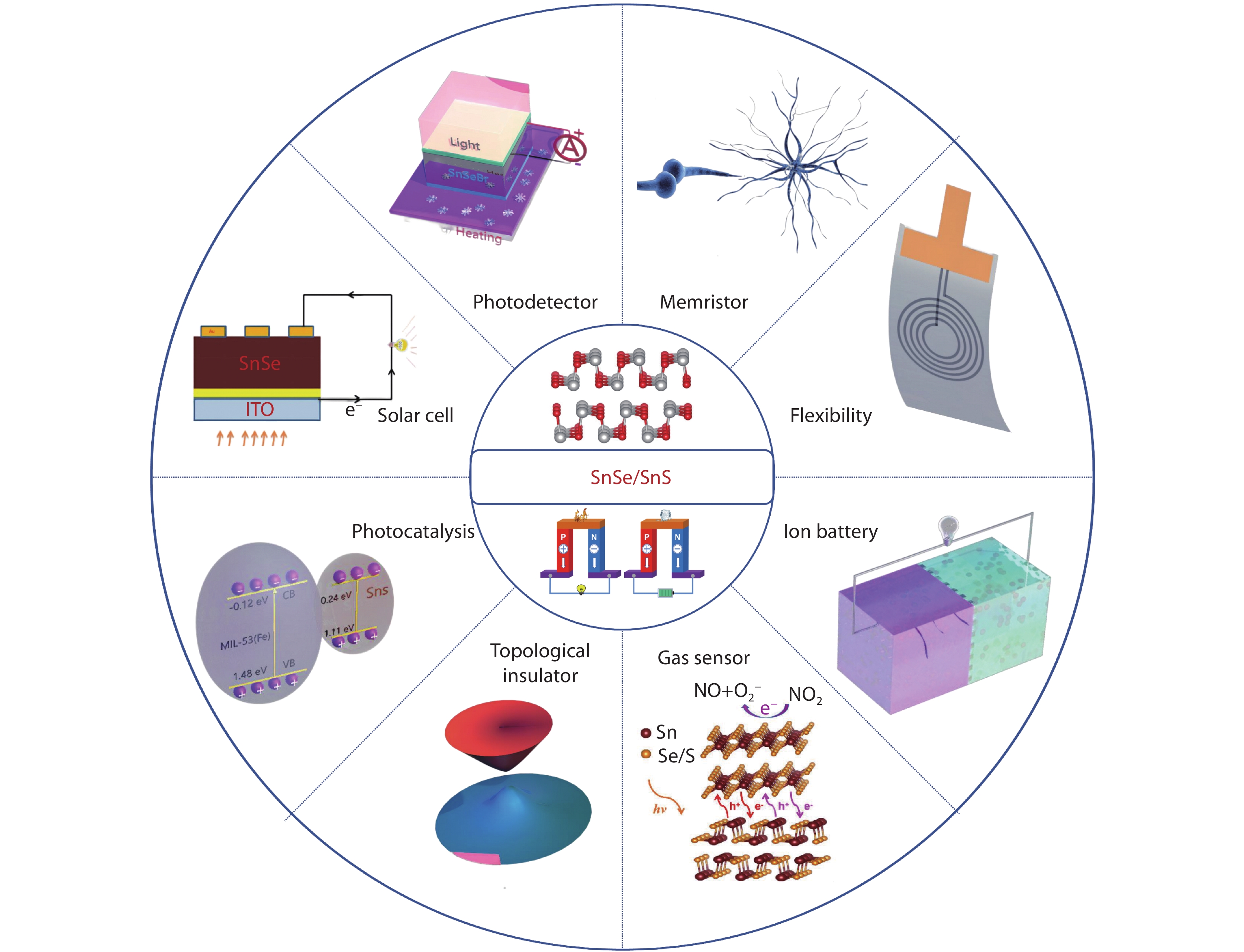
Citation: | Dongrui Liu, Bingchao Qin, Li-Dong Zhao. SnSe/SnS: Multifunctions Beyond Thermoelectricity. Materials Lab 2022, 1, 220006. doi: 10.54227/mlab.20220006 |
Miniaturization, lightweight and highly integration have gradually become the main trends in the development of modern science and technology. Two-dimensional (2D) SnSe/SnS-based materials have recently received widespread attention in the field of thermoelectricity because of the remarkable physical transport properties. However, the peculiar crystal structure also ensures that SnSe and SnS materials can meet the requirements of the miniaturized and highly integrated functional devices, which make them the most notable interdisciplinary hotpots. In this review, we initially analyzed the basic physical properties and outlined the important achievements in thermoelectric field of SnSe/SnS. With the development of preparation technology for thin-film materials and nanomaterials, SnSe/SnS has been successfully utilized in multiple fields, including photothermal, photoelectric and ferroelectric fields. We then elaborated the multifunctions in SnSe/SnS, such as solar cells, photodetectors, photocatalysis, etc. Eventually, some personal summaries and prospects are demonstrated, which might highlight the importance of multifunction and promote the potential applications of 2D materials including SnSe/SnS.
The scientific knowledge system has the characteristics of integration, and the multidisciplinary sciences have always been the growing points of major scientific discoveries and new scientific frontier. So as to cater to the development of science and technology, functional materials which are low-cost, green-energy, easy to synthesize and competitive in specific fields have gradually become the research hotspots. Multifunctional materials, as the name suggests, can be properly applied in various fields, which depend on their excellent electronic, optical, thermal and magnetic properties. Exploring new and highly effective materials with multifunction and establishing their appropriate synthesis methods will definitely promote the progress and development of materials science and technology.
Two-dimensional (2D) materials display very unique properties from three-dimensional (3D) materials because of the quantum confinement effect along the interlayer direction. As popular IV-VI 2D materials, the structure of layered SnSe/SnS originates from a 3D separation of the rock-salt structure.[1] SnSe/SnS possesses excellent chemical stability, thermodynamic stability, tunable band gap and large optical absorption coefficient, and can absorb almost the whole visible-light spectrum.[2, 3] In the past years, SnSe/SnS was originally used as absorbing materials for solar cells.[4-16] Recently, owing to strong anharmonicity and excellent in-plane electrical transport properties, they have gradually emerged and become the most famous materials in the field of thermoelectricity. In 2014, p-type pristine SnSe was found to possess intrinsic low thermal conductivity,[17] and high values of the thermoelectric figure of merit ZT ~ 2.6 ± 0.3 at 923 K in the b-axis direction. Subsequently, 3D-charge/2D-phonon transports and multiple valence bands structure were then discovered, which led to exceptional thermoelectric properties in n-type and p-type SnSe crystals, respectively.[18, 19] In 2021, the average ZT approaching ~1.9 was achieved in p-type SnSe by alloying small quantities of Pb, originating from the momentum and energy multiband synglisis, which might open new era of p-type SnSe crystals as potential candidates for both power generation and thermoelectric cooling.[20] Wide bandgap p-type SnS was also often neglected in thermoelectric field because of its poor electrical conductivity. However, it was then established as thermoelectric candidate since the single-crystal samples were successfully prepared with a two-step method.[21] In 2019, through balancing the competition between the effective mass and carrier mobility by alloying Se, ZTmax ~1.6 at 873 K with ZTave ~ 1.25 at 300-873 K were obtained in SnS0.91Se0.09 crystals.[22]
After proper doping and energy band engineering, SnSe and SnS might possess excellent electronic and optical properties, which broaden the potential applications in other fields in addition to thermoelectricity and solar cells. The currently established multifunction mainly includes photodetectors, sodium-ion/lithium-ion batteries, supercapacitors, photocatalysis, memories, memristors, and topological insulators. Flexible devices have also been developed to meet complex practical application conditions. These multifunctional applications in numerous fields greatly will reflect the research and application values of the SnSe/SnS materials in the future.
Therefore, starting from the basic characteristics of materials, this review studied the intrinsic characteristics of SnSe/SnS, such as crystal structures, electronic structures, phonon structures, and basic physical properties. On the basis of summarizing the research progress of thermoelectricity, we comprehensively analyzed and summarized the typical applications and research progress in other fields, as schematically shown in Figure 1, and combed the logical relationships between the physical characteristics and practical applications of SnSe/SnS. According to the discussion above, we finally summarized and forecasted the future researches and applications of SnSe/SnS in various fields, which might be the guidance for the future development in multifunctional materials, especially for 2D materials in the interdisciplinary sciences.
SnSe/SnS are both crystallized in the layered orthogonal structure with Pnma space group at room temperature, which can be regarded as a distorted rock-salt phase. Around 750 K the crystal structure of SnSe is transited from Pnma space group (Figure 2a) to Cmcm space group (Figure 2b),[31-33] with the calculated band gap changes from 0.61 eV to 0.39 eV, [34] and the lattice constants change from a = 11.49 Å, b = 4.15Å, c= 4.44 Å to a = 4.31 Å, b = 11.71 Å, c = 4.42 Å.[35] The crystal structure of Cmcm phase has higher symmetry than that of Pnma phase. Besides, each Sn (Se) atom bonds to the other three Se (Sn) atoms by sp2 orbitals, and the 5s2 lone pair electrons of Sn stereoscopically exist between four longer van der Waals bonds (Figure 2c). In Figure 2a, each Sn atom is connected with three adjacent Se atoms by strong chemical bonds in the in-plane direction, and the other four Se atoms are connected by weak van der Waals forces, with three in the out-of-plane direction and one in the in-plane direction, which leads to strong anisotropy and giant anharmonicity. SnS has a crystal structure similar to SnSe, i.e., Pnma phase at room temperature and Cmcm phase at high temperature. The similar crystal structure determines that SnSe and SnS have the similar basic transport properties, energy band structures and optical properties. Recently, The new nanometric cubic binary phases SnS[36] and SnSe[37] were reported to have different band gaps and crystal structures from the orthogonal phases, and better transport properties and optical properties were expected.
Carrier mobility is a critical parameter affecting the performance of devices.[38-41] The intrinsic SnSe is a p-type semiconductor owing to the existence of Sn vacancy. The effective mass of SnSe along the a-axis is greater than that along the b/c-axis, indicating that the electrical transport performance in SnSe along the in-plane direction is better than that in the out-of-plane direction.[42] In fact, the main carriers (holes) of p-type SnSe tend to be in-plane two-dimensional (2D) transport, and the main carriers (electrons) of n-type SnSe can carry out three-dimensional transport because of the overlap of conduction band electron orbits in the out-of-plane direction in the process of continuous phase transition.[19] Although undoped SnSe reaches an extremely high ZT value, the practical thermoelectric application requires high ZT values over a wide temperature range. Therefore, further attention was paid to optimize the electrical transport performance of SnSe in low- and mid-temperature ranges. Appropriate dopants can effectively enhance the hole carrier concentration and promote the Fermi level deeper into more valence bands so as to concurrently improve the electrical conductivity and Seebeck coefficient. Zhao et al.[18] proposed that hole doping by Sodium can promote the multiple valence bands transport, leading to the largely enhanced electrical conductivity to >
Single-crystal SnSe is not as adaptable as polycrystal SnSe in the fields of solar thin films and flexible devices due to its low yield, harsh growth conditions and strong rigidity. Due to the grain boundary scattering, polycrystals have much lower carrier mobility, resulting in lower electrical conductivity less than one fifth of that of single crystals.[43] So far, Li, Na, K, and Ag are effective dopants for SnSe polycrystals to improve the electrical transports.[44]
Due to the strong anharmonicity caused by the different bonding modes in the out-of-plane and in-plane directions, SnSe has intrinsic low thermal conductivity. Weak bonding in out-of-plane direction can strongly hinder the phonon transport. The average number of Grüneisen parameters is ~ 4.1 for a-axis, ~ 2.1 for b-axis, and ~ 2.3 for c-axis.[17] The Grüneisen parameter is a reflection of the strength of lattice anharmonicity, and the different values indicate the thermal conductivity of a-axis is lower than that of b, c-axis. In addition to the peculiar crystal structure, a large number of intrinsic off-stoichiometry defects act as phonon scattering centers, further decreasing the thermal conductivity.[45] The lattice thermal conductivity of single crystal SnSe along a-axis is as low as ~ 0.2 W m−1 K−1 at 973 K.[43] The phonon transport properties of polycrystalline SnSe are quite complex. The oxidation degree of the sample is another key parameter in the preparation process.[46-48] The existence of oxide SnO2 greatly improves the thermal conductivity of SnSe. And experimental researches further revealed that controlling stoichiometric ratio[43] and removing surface oxides can substantially decrease the thermal conductivity and enhance the thermoelectric performance of polycrystalline SnSe.[49, 50]
The efficiency for electronic devices is bound up with the energy band gap. For photoelectric applications, SnSe has proper band gap with good photoelectric properties. It also has a high light absorption coefficient, which is very suitable as the absorption layer of solar cells. The shape, structure and preparation methods of SnSe will lead to different band gaps of bulk SnSe, thin film SnSe and nanostructure SnSe.[51, 52] As show in Figure 3c-3e, the band gap of SnSe films is interrelated to the synthesis methods and the number of layers. The energy band gap of SnSe nanostructures is bound up with the grain size owing to the quantum confinement effects,[53] and the band gap raises with the reduction of the grain size at nanoscale.[31]
Therefore, we can adjust the band gap of SnSe through the synthesis methods and controlling the grain size. The thin layer with a thickness of 300 nm can absorb most available spectra. In the visible region, the optical absorption coefficient of SnSe can reach 105 cm−1,[14] which is helpful to absorb more solar radiation and produce electron hole pairs.
SnS is also a p-type semiconductor thanks to the intrinsic Sn vacancy. Sn on S anti-site defect is another adverse defect that affects the carrier concentration and carrier mobility, and the formation of this defect can be inhibited under the growth condition of S-rich. The electrical conductivity of SnS in the 2D plane is much higher than that in the out-of-plane direction due to its layered structure. The carrier concentration of undoped SnS is ~ 1015-1018 cm−3 and the electrical conductivity is only less than 10 S cm−1 at room temperature.[55, 56] Element doping, alloying and full-size hierarchical architectures are helpful to improve the electrical properties of SnS. After Na doping, the electrical conductivity of single-crystal SnS can be elevated by two orders of magnitude at 300 K, and the carrier concentration reaches ~ 1019cm−3 in the whole temperature range.[57, 58] SnS has similar energy band structures with SnSe, with multiple valence band maxima (Figure 4a). The energy gap between the maximum value Γ-Z of the first band and the maximum value U-R of the second band is only 0.056 eV. The Fermi level can easily cross such a small energy difference through hole doping. With the increase of hole carrier concentration, the Fermi level continues to move deep into the valence band. The third and fourth band maxima are located in the Γ-Z and Y-Γ directions, respectively. When the carrier concentration reaches ~ 4 × 1020 cm−3, the fourth valence bands are activated to promote multiple valence bands to participate in carrier transport. Generally, the mobility of SnS polycrystals is lower than that of single crystals, because carriers are strongly scattered by grain boundaries. SnS is an analogue of SnSe, and the similar layered structure makes SnS also with intrinsic low thermal conductivity. Figure 4b shows the high symmetry points marked in the Brillouin zone. The theoretically calculated energy band structure was also be verified experimentally by Angle resolved photoelectron spectroscopy (ARPES) in Figure 4c-4d.
In fact, bulk SnS was rarely used in electronic devices, so researcheres paid more attention to the energy band structure of nanostructures and few layers in SnS. Tritsaris et al.[59] proved the energy band differences of SnS for different layers (2.72 eV, 1.57 eV, and 1.07 eV for single layer, double layer, and bulk SnS, respectively) by the first-principles calculations. Xin et al.[60] used hybrid functional calculations with spin-orbit coupling and further verified that the decrease of band gap with the increase of the number of layers is not monotonic due to the odd even quantum confinement effect. Through theoretical calculations, it was further proved that SnS has a tunable band gap, which can meet the optimal energy band gaps of 1.1 eV ~ 1.6 eV, and the optical absorption coefficient higher than 104 cm−1 can be expected.[56, 61, 62] It is superior to most of the common light absorbing materials, such as CdTe, Si, etc. Therefore, considering the tunable energy band and optical absorption coefficient, SnS is an excellent optical absorption candidate material.
In summary, single-crystal SnSe and SnS possess high in-plane carrier mobility, tunable band gap and high absorption coefficient, which are the important characteristics of multifunctional materials. In recent years, theoretical calculations on SnSe/SnS monolayers showed that they have great carrier mobility along the in-plane direction. More properties and potential applications of single-layer and few-layer SnSe/SnS materials are being explored.[42, 52, 59, 60, 63-68] Enhancing the electrical properties of polycrystals and the mechanical properties of high-performance single crystals will further broaden and promote the potential applications for 2D SnSe/SnS materials.
At first, SnSe and SnS are not suitable for thermoelectric field because of their poor electrical properties, and they are more used in solar cells and photoelectric fields thanks to appropriate band gap and optical properties. Since the high-performance SnSe crystals was discovered in 2014,[43] people have gradually realized that undoped single-crystal SnSe has extraordinarily low thermal conductivity (< 0.4 W m−1 K−1 at 923 K) along the out-of-plane direction because of its strong anharmonicity from the unique 2D crystal structure. At present, the proposed strategies to enhance the thermoelectric figure of merit ZT include energy band engineering,[69-71] full-size phonon scattering,[72] introduction of nanostructures[73] and microstructure manipulations.[74, 75] After the optimization of the above strategies, the maximum ZT of n-type single-crystal SnSe has reached ~ 2.8 at 773 K.[19] Several studies have confirmed that the decoupling of electrical and thermal transports can be realized by modifying the energy band structure (Figure 5a shows the multiple available valence bands and conduction bands available of SnSe) and full-scale phonon scattering engineering in SnSe. Zhao et al.[18] improved the power factor of p-type SnSe by doping Na (~40 μW cm−1 K−2 at 300 K). On the one hand, Na increases electrical conductivity from 12 S cm−1 to >
As an analog of SnSe, the thermoelectric properties of SnS become a focus to be increasingly noticed. Intrinsic SnS with poor electrical conductivity was not considered to be an excellent thermoelectric material. However, it has been proved both experimentally and theoretically that SnS also has intrinsic low thermal conductivity owing to the strong anharmonicity. Therefore, appropriate doping can effectively enhance the thermoelectric properties of SnS.[78, 79] He et al.[22] introduced Se on the basis of SnS doped with 2% Na. With the increase of temperature, the energy bands were gradually sharpened, and the introduction of Se further sharpens the energy bands, which reduce the effective mass and improve the carrier mobility. Compared with undoped SnS, the introduction of Se reduces the energy gap between VBM 1 and VBM 3, increases the band degeneracy and improves the Seebeck coefficient (Figure 5d). As a result, ZTmax ~ 1.6 at 873 K and ZTave ~ 1.25 from 300 to 873 K were obtained. The excellent thermoelectric properties and the reserves of S element abundance further verify the development potential of SnS. The efficiency of SnS-based thermoelectric devices can be further enhanced by jointly improving the performance of p-type and n-type SnS. He et al.[80] proposed that Br doped SnS crystal can realize high-performance n-type behavior. Although the carrier concentration after Br doping is still low as ~ 4.46 × 1017 cm−3, this lower carrier concentration reduces ionized impurity scattering and causes the high carrier mobility approaching ~
Solar cell is a semiconductor equipment that applies solar energy to generate electricity, which directly converts light into electric energy by photovoltaic effect without thermal process. Thin-film techniques of novel generation of solar cells which satisfy the requirements of low cost, high efficiency and environmental protection have captured growing attention. The corresponding efficiency of commercial Cu(In, Ga)Se2 and CdTe absorbing materials are 20.9% and 20.4%,[5] but In and Ga elements are expensive, toxic and polluting. Whereas, it remains a significant challenge to synthesize homogeneous and pure single-phase Cu2ZnSn(S, Se)4, Sb2Se3, CuSbS2 and CuSbSe2 materials with non-toxic and environmentally friendly. 2D SnSe and SnS thin films have gradually applied as absorption materials for solar cells due to the characteristics of easy preparation, environmental protection, non-toxicity, low cost, outstanding optical and electronic properties. On the basis of Shockley-Queisser criteria, the limiting efficiency of SnSe and SnS-based photovoltaic cells reach 32% and 33% respectively. Although, there is a sketchy calculation, showing that the actual efficiencies of SnSe and SnS-based sollar cells is less than one tenth of the theoretical values. The differences between theoretical values and experimental values can be attributed to two major factors: firstly, depositing high purity, compact and non-porous single-phase thin film materials is difficult, and yet to be inadequately resolved; secondly, inappropriate n-type buffer layer causes the band-off.
In 1990, J. P. Singh et al.[81] initially prepared the SnSe heterojunction solar cells with efficiency of 2.3%. Over the past few years, abundant researches were conducted on thin film SnSe solar cells.[10-13, 15, 16, 82, 83] And then, it was found that the most efficient device was p-SnSe/n-Si heterojunction solar cells, which has exhibited an efficiency of 6.44%.[13] In the field of SnS-based solar energy, the first study was reported in 1998.[84] But the transfer efficiency of most SnS-based solar cells has not exceeded 4%.[4, 5, 9, 56, 85, 86] Recently, massive researches have revealed that annealing after film deposition is a shared strategy to improve solar energy efficiency. Annealing contributes to promote grain growth and reduce defect density, which reduces hole-electron recombination, dark saturation current density and shunt conductance.
As show in Figure 6a, Dipak V. Shinde et al.[15] constructed thin film CdS-SnSe heterojunction solar cells, with SnSe film as absorption layer and CdS as n-type buffer layer. Firstly, CdS and SnSe films were deposited on Indium tin oxide (ITO) substrates by chemical bath deposition method, then annealed in air at 573 K for 30 min. Finally, Au electrodes were deposited on the top of SnSe films. The films were well contacted with each other and uniformly deposited on ITO substrate, which avoids the leakage current at the heterojunction interface and effectively inhibits the recombination of electron-hole pairs. The efficiency of solid-state ITO-CdS-SnSe-Au solar cells reached 0.8%. In order to further elevate the efficiency of CdS-SnSe heterojunction, they constructed ITO-CdS-SnSe-polysulfide-Pt-FTO liquid junction cell, and the solar energy conversion efficiency achieved 1.4% due to the existence of polysulfide reduced the loss caused by carrier recombination.
The annealing process can also be applied to SnS-based solar cells. The power conversion efficiency of TiO2/SnS heterojunction solar cells prepared by SnCl2-TU (thiourea) complex solution has reached 4.8%.[8] High purity orthogonal SnS was prepared by spin-coating SnCl2-TU solution on ns-TiO2/TiO2 blocking layer (BL)/fluorine-doped tin oxide (FTO), annealing in Ar atmosphere for 3 min at 375 °C, and then depositing Au electrode to form FTO/BL-TiO2/ns-TiO2/SnS/Au. Figure 6b shows that cross-sectional image of SnS-based solar cells observed by FESEM (field emission scanning electron microscope). The efficiency of solar cells reached 3.9%. The annealing process ensured the orthorhombic phase SnS with high purity, which prevented the Au electrode from directly contacting ns-TiO2 or FTO. And the reason for short circuit of the device was the inferior crystallization of SnS. Through additional SnCl2 post-treatment, the efficiency reached 4.8%. Excessive SnCl2 helps SnS to form a smooth and dense film, because Cl-1 was introduced into SnS lattice to decrease lattice defects.
In the future, more researches should enhance the properties of SnSe/SnS-based solar cells through optimizing the material preparation methods, modulating the lattice mismatch of composite materials, and selecting appropriate n-type buffer layers.
Lithium-ion batteries (LIBs) and Sodium-ion batteries (SIBs) are regarded as a green energy storage device to replace fossil energy. During charge and discharge, LIBs/SIBs utilized Li+/Na+ to be repeatedly embedded and removed in the positive and negative electrodes to transfer charges and generate electric energy. High energy density and long cycle life are important parameters to evaluate the performance of batteries. Despite that LIBs have occupied a large market share, low-cost SIBs have more development prospects.[87] Comparing with LIBs, poor cycle performance and insufficient charge capacity are the fatal problems of tin-based SIBs anode materials. What is surprising is that 2D SnSe/SnS with unique electronic properties can solve these problems. SnSe/SnS-based electrodes allow more Na+ to be stored, and the theoretical charge capacity can achieve
As shown in Figure 7a, Ren et al.[115] proposed to directionally grow vertical SnSe nanoplates on N-doped graphite by the cation-exchange method. Meanwhile, the first-principles calculations indicated that N-doped graphite is beneficial to enhance the Sn-C bonds between SnSe and N-doped graphite. Strengthening Sn-C bonds accelerates the transfer of electrons between graphite layer and SnSe layer and the diffusion rate of Na+ between SnSe sheets, which also prevent the aggregation of SnSe nanoplates during multiple cycles process. Accordingly, the SnSe nanocomposite electrodes exhibit large capacities of 723 mA h g−1 at 25 mA g−1, and capacity retention of 82% for over 200 cycles at 2 A g−1.This study has promoted the development of the high-performance SIBs.
Xiong et al.[116] deposited SnS nanoparticles (NPs) electrodes on chemically modified graphene to form composite electrodes, which improved the conductivity of the electrodes and offered SnS a larger phase transition buffer space between layered graphite (Figure 7b). Thus, the composite anode indicates excellent capacity retention rate of 87.1% for
At present, compared with LIBs, SIBs have no great advantages except that the reserves of sodium are richer than lithium-ions. Therefore, the optimization of the performance of sodium-ion batteries is far from enough.
With the development of renewable energy such as solar energy, it is extremely urgent to develop efficient energy storage equipment. Supercapacitors are energy storage device by separating charges on the interfaces between electrode and electrolyte. Compared with ion batteries, supercapacitors have greater power density and longer cycle life.[117-122] Nanostructured electrode is an ideal electrode material, which can decrease the diffusion length of ions/electrons and increase the active sites and alleviate the volume change of electrode.[123, 124] The optimization strategy of supercapacitor electrode material is alike to that of LIBs/SIBs. So far, the common electrode materials are metal oxide,[125-129] 2D metal sulfide nanomaterials,[130-133] carbon/carbide nanomaterials[134-138] and so on. In recent years, SnSe and SnS are gradually emerging in the field of supercapacitors because of unique electronic properties and redox reversibility.
Ni et al.[139] suggested that a simple way for microwave-assisted synthesis of SnSe electrode. As shown in Figure 8a, the tin source solution and selenium source solution were mixed and stirred, and then heated in a microwave oven for 15 minutes to form SnSe particles. Compared with the previous hydrothermal synthesis of SnSe electrode material,[140] microwave assisted synthesis can quickly transfer microwave energy to the reaction mixture to realize internal heating and rapid nucleation. It has the advantages of energy saving and uniform heating.
Himani chauhan et al.[141] synthesized surfactant-free SnS nanorods by solvothermal method , which can be used as candidate electrode materials. As can be seen from Figure 8b, Liu et al.[27] reported that SnS composite graphene formed 3D porous electrode, which inhibits the re-aggregation of SnS after multiple cycles. This report offers a novel idea for improving the performance of 2D electrode materials. In the following researches, improving the energy density of capacitors and maintaining the high compatibility between electrodes, electrolytes and device systems are the core development direction.
Recently, extensive studies have shown that memristor is most likely to become the core electronic component of artificial intelligence according to its working principle. Memristors can simulate the process of learning, memorizing and processing information of human brain by continuously adjustable conductance, but the core issue is to seek materials that can store prodigious information and process information efficiently. At present, oxides, sulfides and organic compounds are mainly involved in memristors,[142-144] and utilizes oxygen vacancies, silver-ions and copper-ions to simulate ions diffusion in neurons.[142, 145-148] With the improvement of the requirements for the running speed of memristors and information storage capacity, the device is gradually developing towards miniaturization and integration. In the out-of-plane direction, the 2D material is connected by van der Waals forces. According to this characteristic, the excellent properties of 2D memristors can be hardly influenced when reducing the volume of the devices. Excitatory and inhibitory postsynaptic potentials are two basic synaptic responses of human nervous system under different functions. Recent studies have pointed out that the co-release of glutamate and GABA neurotransmitters at the end of a single axon in the ventral tegmental area can lead to the reconfiguration of postsynaptic potential between excitability and inhibition. As shown in Figure 9a-9b, He et al.[149] prepared black phosphorus-SnSe heterojunction to simulate the reset process of postsynaptic potential after synaptic simultaneous release of excitatory and inhibitory transmitters. The appropriate bandgap of black phosphorus forms the heterostructure with SnSe, resulting in tunable rectifying electrical characteristics. In addition, the charge transfer between the natural oxide of black phosphorus and black phosphorus channel is used to realize synaptic behavior, which can be reconfigured between excitatory and inhibitory responses. This potential reconfigurable artificial synaptic device can simplify the device structure and can be applied to artificial neural networks in the future. Subsequently, they[150] applied Markov chain algorithm to realize the machine learning on SnOx/SnSe/SnOx heterojunction devices. Wang et al.[151] suggested that high-performance Pd/SnSe/NSTO devices can simulated the transfer of Na+, K+, Ca2+ between synapses of human neurons by the formation of Pd filaments and the diffusion of Pd2+ in SnSe films, and realized simple algorithm operation.
Recently, Au/SnSe/graphene/SiO2/Si two-terminal memristors has been demonstrated to be a candidate material for simulating human neural network.[152] In Figure 9c, the memristors are realized by intrinsic Sn vacancies in SnSe. Au electrodes and graphene electrodes were used as presynaptic and postsynaptic, respectively. SiO2 is used as an insulating layer to prevent current leakage. The high thermal conductivity of graphene can enhance the thermal diffusion performance of memristors. Under the external electric field, Sn vacancies are arranged into conductive filaments connected with Au and graphite electrodes, indicating that the storage resistance is in low resistance state (SET). When reverse voltage is imposed, Sn vacancy conductive filaments are broken and memristors are in high resistance state (RESET). The memristors has the characteristics of stability, uniformity, high density storage and low power, which are the best device so far to realize the function of biological synapse.
The main sources of NO2 and SO2 emissions are fossil fuel combustion, automobile exhaust and industrial production, which not only cause enormous harm to the natural environment, but also causes serious damage to human respiratory system and kidney.[153, 154] A challenging problem which arises in this domain is to develop devices for detecting NO2 and SO2. In previous studies, it has found that metal oxides and metal oxide heterojunction gas sensors have lots of disadvantages, such as, undistinguished gas selectivity and high operating temperature.[155-161] 2D materials can effectively solve the above problems due to their thin atomic layer and large specific surface areas. Gas molecules (NO2, SO2, etc.) can be adsorbed on the film substrate and transfer electrons to change the band structure, which can influence the electronic properties of the material (such as conductivity) and can be detected immediately. Graphene, phosphorene and MoS2 have been proved to be applied as gas sensors experimentally and theoretically.[162-167] However, the poor stability of phosphorus in air limits its further development.[168]
Recently, the first-principles calculations have revealed the feasibility of adsorption of NO2 and SO2 on SnSe and SnS monolayers.[169-172] Theoretical calculations showed that SnSe and SnS have moderate adsorption energy and large charge transfer for NO2 and SO2 molecules. Recently, Wang et al.[173] synthesized p-SnSe/n-SnSe2 2D heterojunction by one-step colloid method to detect NO2 gas. At room temperature, pure SnSe2 has poor gas adsorption capacity and conductivity, which affect the sensing response. As a result of narrower band gap and superior intrinsic conductivity, SnSe can promote the adsorption capacity and conductivity of SnSe2 at room temperature. And the SnSe(50%)/SnSe2(50%) heterojunction exhibits n-type conductivity. As shown in Figure 10a, when the SnSe2 part of the heterojunction contacts NO2 molecules, the following reactions occur:
2NO2(g)+e−(hν)→2NO(hν)+O−2(hν) |
Additional electron-hole pairs are produced at the heterojunction interface when illumination is applied. In Figure 10b, the electrons of SnSe2 react with NO2 molecules, which destroy the balance of PN junction and widen the width of built-in electric field. The increase of built-in electric field width and the decrease of electron concentration in heterojunction lead to the increase of resistance and improve the detection ability of NO2 gas. When 405 nm laser irradiation and 0.1 ppm NO2 gas are applied simultaneously, the response performance of the gas sensor has reached 103% due to the additional photogenerated electrons react with NO2 gas on the surface. Sun et al.[174] suggested a high performance SnS2/SnS p-n heterojunction NO2 gas sensor, and the sensing response value was 660% at 4 ppm NO2. In addition to acting as a gas detector, M. F. Afsar et al.[175] reported SnS nanosheets as sensors for volatile compounds alcohol and propanol. The feasibility of SnSe/SnS as detectors for other harmful gases and volatile compounds is still a valuable research direction.
Modern information storage and processing technology are accomplished by magnetic moment and carrier transport in magnetic materials. Metal and ferrite ferromagnetic materials do not possess semiconductor characteristics, and the Curie temperature is low, in which the magnetism has disappeared in practical applications at room temperature. Semiconductor materials with high mobility and signal processing are incompatible with ferroelectric and ferromagnetic properties. Therefore, doping magnetic elements into non-magnetic semiconductors can form diluted magnetic semiconductors with ferromagnetic, ferroelectric and ferroelastic properties. Through this method, some semiconductor materials with superior performance can also be used for non-volatile memories. B. Parveen et al.[176] doped magnetic Ni in SnS nanocrystals to prepare diluted magnetic semiconductor, which showed ferromagnetism at room temperature.
Ferroelectric tunnel junctions have a heterostructure with ferroelectric ultrathin film as barrier layer and electrode lead sandwiched on both sides, which have tunnel electrical resistance effect. It solves the problems of short service life, limited reading time, low storage capacity and difficult miniaturization of traditional ferroelectric memories. It has a wide application prospect in nonvolatile memories. In many ferroelectric tunnel junctions, 3D lattice materials are used as the barrier layers. But with the reduction of the material size, the film will appear depolarization effect and the ferroelectric property will disappear.[177, 178] Therefore, it is difficult for the 3D ferroelectric tunnel junctions to minimize the device size.[177, 179-181] 2D materials with ferroelectric properties break the critical thickness problem of three-dimensional materials and greatly reduce the size of devices.[182-188]
Wu et al.[64] predicted through the first-principles calculations that SnSe and SnS with high mobility and anisotropic physical properties can also have ferroelectric and ferroelastic properties, and have high Curie temperature, which is expected to maintain the ferroelectric properties of materials at room temperature. Shen et al.[25] doped In and Sb elements in SnSe to prepare In: SnSe/SnSe/Sb: SnSe ferroelectric tunnel junctions (see Figure 11a in detail). Compared with the previous ferroelectric tunnel junctions, this report used pure SnSe as the ferroelectric barrier, and uses In-doped p-type SnSe and Sb doped n-type SnSe as the two ends of electrodes respectively. The ferroelectric tunnel junctions with the same structure can avoid the lattice mismatch and reduce the difficulty of device preparation. Since the polarization of SnSe in the plane is the same along the b- and c-axis, in Figure 11b, they defined the polarization directions as P+x and P-x, and the original width of the 2D ferroelectric barrier is d. When the polarization direction is P+x, the left side of the ferroelectric barrier is negative and the right side is positive. Electrons and holes gather on both ends of the ferroelectric barrier, and the width of the ferroelectric barrier becomes d1. When the polarization direction is P-x, the electrons and holes on both ends of the ferroelectric barrier are neutralized, leaving only ionized donors and acceptors, and the ferroelectric barrier width becomes d2(d1 < d2) (Figure 11c). In the direction of P+x polarization, the carriers converge at both ends of the ferroelectric barriers, which make the aggregation zone conductive and reduces the effective barrier width. In the direction of P-x polarization, the carriers are consumed at both ends of the ferroelectric barriers, and the interface region changes from a conductive state to an insulating state, increasing the width of the effective barrier. As show in Figure 11c, the average barrier height in two polarization directions is φR and φL. Because the conductivity of tunnel electrons is affected by the height and width of the barrier, the resistance in the P+x polarization direction is small, which is regarded as the "ON" state, while the resistance in the P-x polarization direction is large and is called the "OFF" state. In the previous ferroelectric tunnel memories, only the height of ferroelectric barrier is changed when the polarization direction changes. However, the memories reported in this paper utilized the unique properties of semiconductor materials to achieve high tunneling electroresistance effect of
Phase change memories (PCM) are other devices to realize the nonvolatile memory. The storage state is determined by the resistance value of the resistor, and the data are stored by the resistance difference in mutual transformation of crystalline state and amorphous state. Campbell et al.[189] verified the potential of Ge2Se3/SnSe device as phase change memories.
Photodetectors are devices thattransfer optical radiation signals into electrical signals. According to its absorption spectrum, photodetectors can be applied in many fields such as commerce, military and so on. So far, however, there was little research about a wide spectrum with detection range from infrared to ultraviolet in photodetectors. The appearance of 2D materials brought a breakthrough for the development of photodetectors. Compared with the traditional semiconductor materials, they have high in-plane mobility, stable chemical properties, good mechanical flexibility, exceptional photoelectric conversion properties. More importantly, there is no chemical bonds in the vertical direction of the plane, which is convenient for constructing 2D heterojunction and no-dangling bonds interlayer structure.[190-192] Therefore, 2D materials may become candidate materials for the next-generation flexible optoelectronic devices. Graphene is widely used in infrared photoelectric detection, but graphene has zero-band gap and low absorbance, which are easy to oxidize and adsorb impurities.[193] The features of transition metal chalcogenides (TMDs)-based photodetectors (MoS2, WS2, and WSe2) are high photo-responsiveness and photo-sensitivity, however, they are only sensitive to visible light and weak to infrared light.[194-196] Comparing with graphene and transition metal chalcogenides, SnSe/SnS possess high absorption coefficients and tunable band gaps, which are expected to be candidate materials for wide spectrum photodetectors.[197, 198] When 2D semiconductor materials are used alone, their electronic and optical properties often cannot meet the application conditions of photodetectors. Previously, the traditional optimization strategy was to improve the synthesis methods of SnSe/SnS thin films and nanocrystallines[31, 52, 140, 199-210], or composite other materials.[211-216] In recent years, researches provided new insights to improve the performance of SnSe/SnS-based photodetectors through superimposing a variety of effects on traditional optoelectronic devices.[206, 209, 211, 217-221] For example, photoelectric effect is coupled with photothermal effect,[217, 220, 222-225] piezoelectric effect[226-228] and ferroelectric effect.[229, 230] Whereas, the large band gaps of ferroelectric materials narrow the spectral detection range, the photothermal effect cannot continuously enhance the photoelectric signal, and the piezoelectric strain inevitably destroys the device structure. Thus, people began to use thermoelectric effect to couple with photoelectric effect.
Recently, Ouyang et al.[231] suggested an efficient self-powered photodetector using the extraordinary thermoelectric properties of SnSe: Br and SnS: Na single crystals. Figure 12a is the structural diagram of the proposed photodetector. The device structure is ITO/SnSe: Br/Ag. Under the light of 760 nm, ΔT = 1.5 K along the (100) direction is applied to the single crystal SnSe, the light energy and heat energy are converted into electrical signals at the same time. And the most interesting finding was that the output voltage and current are increased by 38.1% and 81.9% compared with those without temperature difference. This study verified that the combined action of thermoelectric effect and photoelectric effect enables to improve the performance of photodetectors. The work functions of ITO and Ag are 4.8 eV and 4.26 eV, respectively, without temperature difference. The electron affinity and band gap of SnSe: Br are 3.4 eV and 0.9 eV. When SnSe: Br contacts with ITO electrode and Ag electrode, the contact surface forms the Schottky barrier. In Figure 12b, under the condition of illumination only, SnSe: Br produces photo-generated electron-hole pairs. Under the action of built-in electric field, photo-generated electrons transfer to Ag electrode and holes transfer to ITO electrode. The current direction is ITO electrode to Ag electrode in the external circuit, and the device forms a closed loop.
As shown in Figure 12d, under the condition of no light, the temperature difference is applied to Ag electrode and ITO electrode, the external circuit current flows from ITO electrode to Ag electrode, which is consistent with the current direction under illumination. The thermoelectric effect promotes the transfer of electrons from ITO electrode (high temperature end) to Ag electrode (low temperature end). Meanwhile, phonons also transfer to the low temperature end, dragging electrons to move rapidly. Both actions accelerate the electron transfer. However, the thermoelectric effect simultaneously causes the energy band bias, which causes the decrease of the Schottky barrier of ITO and SnSe: Br (see the Figure 12e in detail). The increase of Schottky barrier between Ag and SnSe: Br decreases the transfer speed of electrons to Ag electrode and intensifies the recombination of electrons and holes. Thus, the thermoelectric effect suppresses the photoelectric effect. Nevertheless, when light and temperature difference are applied simultaneously, they observed that the directions of photo-current and thermoelectric-current were consistent, and then the current increased, as shown in Figure 12f, which indicates that thermoelectric effect elevates the response ability of photodetectors. Based on the above phenomenon, the thermoelectric effect has two functions: 1) thermoelectric temperature difference and phonon diffusion can promote the transfer of photogenerated electrons. 2) the tilt of energy band shift caused by thermoelectric effect reduces the transfer speed of photo-generated electrons and increases the recombination rate of electrons and holes. In fact, the former played a dominant role. This suggested that the coupling of thermoelectric effect and photoelectric effect improves the performance of photodetectors, and the continuous application of temperature difference will not cause damage to the device. The above two reports also further verified the excellent thermoelectric properties of SnSe and SnS.
In recent years, the environmental problems caused by industrialization have posed a serious threat to global biosafety. Semiconductor photocatalytic technology is a critical part for solving the environmental pollution problems efficiently, which uses solar energy to treat environmental pollutants and decompose water to produce hydrogen.[232-235] It has the advantages of low cost, low energy consumption and less secondary pollution. Photocatalysis absorbs photons through semiconductors and produces photo-generated electron-hole pairs. Some electrons and holes transfer to the surface of the material, and react with surface substances by oxidation or reduction. The other part is electron-hole pairs recombination. In the prevailing view, TiO2, Bi2WO6, and other materials have superior photocatalytic performance, however, the absorption range of visible light is narrow, which hinders the exertion of photocatalytic ability. On the contrary, SnSe and SnS with suitable band gaps and large optical absorption coefficients are expected to be promising photocatalytic materials. In fact, the two materials have not been adequately showed photocatalytic activity, and the recombination rate of photo-generated electrons is fast. Manufacturing SnSe/SnS-based heterojunction is an effective approach to improve the photocatalytic activity. Heterojunction photocatalytic materials can adjust the light absorption range compared with the inherent energy band structure of single-phase materials. By adjusting the energy band structure, the separation of photo-generated carriers can be accelerated and their recombination can be inhibited.[236-238] A. Ghorban shiravizadeh et al.[239] prepared SnSe/rGO (reduced graphene oxide) nanocomposites photocatalytic materials by co-precipitation method. Bi2WO6/SnS heterostructure prepared by Tang et al.[240] through surface functionalization approach showed stronger catalytic activity for Rhodamine B dye than single phase Bi2WO6 and SnS. Xia et al.[241] prepared novel metal-organic framework/stannous sulfide nanocomposite photocatalysts by one-step position process, which can efficiently degrade Cr element under visible light.
As show in Figure 13, Li et al.[26] reported a novel water treatment strategy combining photodegradation and photothermal evaporation. Photothermal effect depends on the recombination of photogenerated electrons and holes to generate heat, while efficient photocatalytic effect needs to suppress the recombination of photogenerated electron-hole pairs. Generally, the two effects cannot be applied in the same device. Li et al.[26] prepared SnSe-SnO2 nanocomposites by exposing SnSe nanoparticles to air at 413 K for 1 h. The outer layer is n-type SnO2 and the core is p-type SnSe. SnSe-SnO2 nanocomposites exhibited excellent photocatalytic and photothermal properties. The surface thickness of SnO2 was less than 5 nm, which reduced the influence on the photothermal effect of SnSe in the core and acted as the active layer of the photocatalysis. SnSe cores performing photothermal effect have large absorption coefficient and visible light absorption range, which promote the photocatalytic effect. Photocatalytic effect of SnO2 is also promoted by photo-generated carriers on the surface of SnSe-SnO2 heterojunction. The photocatalytic mechanism of this device is shown in Figure 13. During the photothermal-photocatalytic production, light firstly promotes the generation of electrons and holes in semiconductor materials. Then, these charges are transferred to the surfaces of the materials and catalyze the reactions with the surrounding substances, while the remaining unreacted electrons will transfer from the conduction band to the valence band and release heat, which can produce the photothermal effect.
As posted before, Bi2WO6 has outstanding photocatalytic performance under visible light irradiation, but its light absorption range is narrow.[235] Li et al.[242] prepared SnS/Bi2WO6 Z-scheme heterostructure by bath sonication method, which can be used as an efficient photocatalytic material for wastewater treatment. The light absorption range of SnS covered almost the whole visible spectrum. So, SnS extended the light absorption range of SnS/Bi2WO6 and hindered the recombination of photo-generated carriers. However, SnS and SnSe nanoparticles tended to aggregate in the photocatalytic process, which led to the decrease of specific surface area and light absorption efficiency. Therefore, it is a current challenge to select materials with large specific surface area, suitable energy band structure and good photocatalytic performance to composite SnSe and SnS.
Since topological insulators were discovered in 2007, they have gradually become the new focus in condensed matter physics. Topological insulators (TIs) are materials with internal insulation that allow charge movement at the interface. In 2011, Fu et al. proposed the concept of topological crystal insulator (TCI).[243] Different from topological insulators, topological crystal insulators are new-type topological material. Its outstanding gapless conductive surface state (topological surface state) is protected by both crystal symmetry (point group, space group, etc.) and internal symmetry (time inversion, chirality, etc.), which reduces the difficulty of improving the topological properties of materials and makes them more promising.[243-245] In recent years, 2D topological insulators with quantum spin effect have attracted extensive attention with the improvement of preparation technology for 2D materials. 2D topological insulators are also called quantum spin insulators. The quantum spin Hall effect was firstly discovered in graphene and HgTe quantum Wells. However, graphene has a small energy gap, and the quantum spin Hall effect of HgTe demands to be realized at low temperature. In order to adapt the actual demand, researchers turned to the semiconductor of IVA-VIA elements. So far, the reported topological crystalline insulators include SnTe and Pb1−xSnxSe/Te.[244-248] PbSe, PbTe and PbS have been predicted to be topological insulators through alloying and applying pressure.[249] Sun et al. verify that SnSe and SnS are intrinsic topological crystal insulators through first principle calculations.[250] This TCI is characterized by an even number of Dirac cones on the highly symmetrical (001), (110) and (111) surfaces, which are protected by reflective symmetry, perpendicular to the (−110) mirror symmetry plane.
Wang et al.[30] proposed to use molecular beam epitaxy technology to prepare SnSe (111) thin films on Bi2Se3 substrate. As Figure 14a, the surface topological state of SnSe had four Dirac cones, which were located in the time reversal invariant momenta of the (111) surface Brillouin region, namely one −Γ point and three −Mpoints, respectively. The results of ARPES (Angle-resolved photoemission spectroscopy) spectra showed that the two energy bands cross to form Dirac cone. According to the calculation results, it was confirmed that the Dirac cone was the surface state. There were even number Dirac cones on the highly symmetric surfaces, which was an obvious feature of topological crystalline insulators (see theFigure 14b-14ein details). The first-principles calculations further verified the above experimental phenomena. At present, most of the relevant articles on topological insulators are about the theoretical calculations of SnSe and SnS, which is still a long way from practical applications.
In the above, other applications of high-performance SnSe/SnS thermoelectric materials have been elaborated. However, the rigid SnSe/SnS are not adequate for the needs of flexible wearable devices. Advances in the performance of novel flexible fiber/textile/film provide a promising path for the development of flexible wearable devices. In recent years, flexible thermoelectric generators, flexible energy storage materials and flexible photodetectors as core devices are in the new frontier of researches.
The electrode materials with superior mechanical flexibility can replace the traditional rigid electrodes as the core components of the flexible energy storage devices and provide continuous energy supply. Xia et al.[29, 251] reported that SnSe/C and SnS/C nanofibers were synthesized by electrospinning and calcination as flexible anode electrodes. Liang et al.[252] fabricated SnS porous films as flexible electrodes for Al-ion batteries. Compared with rigid photodetectors with large thickness and poor mechanical performance, portable flexible photodetectors are more suitable for wearable electronic devices. Recently, attention has been geared toward SnSe/SnS-based flexible photodetectors.[28, 253-255] Zhong et al.[208] indicated that high quality polycrystalline textured films synthesized by one-step chemical method can be applied to wearable photothermoelectric(PTE) detectors. In Figure 15a, different from the traditional photodetectors based on photovoltaic principles, PTE detectors have the function of thermoelectric generator. Flexible thermoelectric devices can be extensively used in various fields because of their high reliability, superior mechanical flexibility and good high temperature stability. A crucial question with regard to flexible thermoelectric material is how to maintain the excellent properties after multiple bending. In 2014, Xu et al.[256] proved that the flexibility of SnS thin films and SnS-based heterojunctions can be realized by using flexible polyimide substrates. Subsequently, Yin et al.[257] also successfully prepared SnSe/SnS thermoelectric thin films through controllable colonial synthesis approach. Nicolas Augustus Rongione et al.[258] reported that SnSe flexible films for waste heat recovery near room temperature were synthesized by heating hydrothermal synthesis temperature difference method and they worked well after
Excitingly, Zhang et al.[260] utilized laser-induced directional crystallization process to synthesize SnSe single crystal fibers (see the Figure 15c in details), which provided a novel idea for the application of rigid high-performance single crystal in flexible devices. The overlength single crystal SnSe fiber with high thermoelectric properties was realized by "two-step method". Firstly, the fiber with SnSe polycrystalline material as the fiber core is prepared by hot drawing process. Secondly, the CO2 laser is used to melt and recrystallize the SnSe core, and the single crystal SnSe structure is realized over the whole fiber length by laser thermal scanning, the ZT value increases significantly from about ~ 0.58 to ~ 2 at 862 K.
In summary, it must be highlighted that materials with multifunction features will definitely become more and more important in the fields of application science and technology. In this review, the multifunction of ten applications beyond thermoelectricity for SnSe/SnS materials has been discussed and the relations between the applications and physical mechanisms were also analyzed above. On this basis, we will finally look forward to the future development directions of the 2D multifunctional SnSe/SnS materials from the perspectives of materials optimization, synthesis methods and devices technology. The following personal prospects might provide certain guidance for next-step study on the applications of SnSe/SnS as well as other 2D multifunctional materials.
1. SnSe/SnS have been the most promising thermoelectric candidates because of their exceptional performance in p/n-type materials, especially for single-crystal samples. However, the poor mechanical properties and processibilities largely limit their applications as components of thermoelectric devices. The full-scale and highly-integrated thermoelectric devices based on p-type SnSe/SnS crystals have just been established, while the power generation efficiency and thermoelectric cooling performance of the devices were still far away from expectation. Besides, the better performance in n-type SnSe/SnS was obtained along the out-of-plane direction, which poses greater challenges for device assemble. Polycrystal SnSe with comparable and even higher performance compared with the single crystal has recently been developed due to the purification of raw materials and surface oxygen removal, but the high performance needs to be further verified and stabilized in normal environment. Therefore, for practical applications of SnSe/SnS thermoelectrics, developing crystal-preparation technology to improve the mechanical properties of single crystals, searching for better contact materials in thermoelectric devices, establishing high-performance n-type counterparts, and stabilizing the high performance for polycrystals will be crucial in the future.
2. It is required to further explore the band off of PN junctions formed by SnSe/SnS and n-type buffer layers, in order to build an appropriate energy band arrangement from substrates to upper materials, and reduce the losses caused by barrier potential in the process of photoelectron transport and dark current as much as possible.
3. At the grain boundary and heterojunction of thin-film solar cells, the existing defects (such as intrinsic Sn vacancy) will accelerate the recombination of electrons and holes in solar devices and cause the increased saturation dark current, which are harmful for the final performance of solar cells. Trying to add annealing process might be the way to solve this problem. As mentioned above, the annealing process can effectively reduce the defects in electrode composites, while the heating process may change the structures or chemical compositions of the materials. It is very vital to seek a process that can decrease the heterojunction interface defects without changing the materials structures and compositions.
4. The strong anisotropy of SnSe and SnS materials not only gives special properties to the materials, but also hinders the growth of thin film samples. Because of the anisotropic growth of SnSe and SnS along the in-plane direction, pinholes are formed when the substrate is exposed, which degrades the performance of solar energy conversion devices. Hence, controlling the growth orientation of thin films is a great technical challenge.
5. Due to the multivalent characteristic of Sn, taking SnS as an example, the synthesis of SnS films will be accompanied by the formations of by-products SnS2 and Sn2S3. Therefore, improving the preparation process of pure SnSe and SnS films is an effective method to realize the theoretical photoelectric conversion efficiency.
6. The electronic devices have gradually been developed from the previous rigid systems to the flexible systems. Due to the rigidity of single crystals, most flexible materials prefer to use polycrystalline SnSe/SnS, but the electrical transport performance of polycrystallines is far inferior to that of single crystals. SnSe single-crystal fibers have been prepared by the directional melting and recrystallization of SnSe core by CO2 laser.[260] This method provides us with a good idea. On the other hand, texturing may also be a breakthrough to promote the electrical transport properties of polycrystalline materials.
7. For energy storage devices, it is required to prepare pure large-area SnSe and SnS energy storage materials, reduce the electrode impurities and increase the contact area with electrolyte. These are essential to improve the charge storage and power density of the energy storage devices.
8. Innovatively, the 2D electrode materials were constructed into three-dimensional electrode materials by compounding other materials, which can effectively prevent the gather or re-accumulation of the electrode materials after multiple cycles, increase the phase transition space during electrode charging and discharging and extend the operating lifetime of the 2D electrode materials.
9. The solid electrolyte interface (SEI) films of negative electrode materials have a double-edged sword for lithium-ion/sodium-ion batteries. On the one hand, the formation of SEI films consumes some lithium/sodium ions, which increases the irreversible capacity. On the other hand, SEI membrane can prevent the co-embedding of other impurities in the electrolyte, and inhibit the damage to the electrodes structures and improve the service lifetime of the electrodes. Therefore, studying the formation mechanisms and properties of SEI can further improve the service lifetime of electrode materials for energy storage devices. Controlling the formation of SEI films also helps to improve the initial coulomb efficiency (ICE) of energy storage devices, which are closely related to the energy density.
10. Heterojunctions are mostly formed from materials with the same crystal structure. It is very difficult to form heterojunctions between SnSe/SnS and other semiconductor materials with different structures. For example, when SnSe/SnS and three-dimensional materials form a composite structure, a large number of dangling bonds will appear in the form of composite centers and scattering centers, which hinders the carrier transport, but this strategy can greatly improve the performance of the devices. Therefore, finding suitable composites is a challenge.
11. Last but not the least, the combination of the multidisciplinary sciences has become more and more crucial in many research fields. Coupling more than one effect (such as thermoelectric and photoelectric effects, etc.) on a traditional electronic device might lead to unexpected performance optimization in each certain field. This will leave broader research directions in the future.
This work was supported by the National Natural Science Foundation of China (
The authors declare no conflict of interest.
The manuscript was drafted by Dongrui Liu and revised by Dr. Bingchao Qin and Prof. Li-Dong Zhao. All authors had approved the final version of the manuscript.
1. | G. Tan, L. D. Zhao, M. G. Kanatzidis, Chem. Rev, 2016, 116, 12123 |
2. | C. Chang, D. Wang, D. He, W. He, F. Zhu, G. Wang, J. He, L.-D. Zhao, Adv. Energy Mater, 2019, 9, 1901334 |
3. | L. Makinistian, E. A. Albanesi, Phys. Status Solidi B, 2009, 246, 183 |
4. | B. C. Qin, L. D. Zhao, Materials Lab, 2022, 1, 220004 |
5. | V. Steinmann, R. Jaramillo, K. Hartman, R. Chakraborty, R. E. Brandt, J. R. Poindexter, Y. S. Lee, L. Sun, A. Polizzotti, H. H. Park, R. G. Gordon, T. Buonassisi, Adv. Mater, 2014, 26, 7488 |
6. | J. Y. Cho, S. Kim, R. Nandi, J. Jang, H.-S. Yun, E. Enkhbayar, J. H. Kim, D.-K. Lee, C.-H. Chung, J. Kim, J. Heo, J. Mater. Chem. A, 2020, 8, 20658 |
7. | P. Sinsermsuksakul, J. Heo, W. Noh, A. S. Hock, R. G. Gordon, Adv. Energy Mater, 2011, 1, 1116 |
8. | H. S. Yun, B. w. Park, Y. C. Choi, J. Im, T. J. Shin, S. I. Seok, Adv. Energy Mater, 2019, 9, 1901343 |
9. | D. Lee, J. Y. Cho, H.-S. Yun, D.-K. Lee, T. Kim, K. Bang, Y. S. Lee, H.-Y. Kim, J. Heo, J. Mater. Chem. A, 2019, 7, 7186 |
10. | G. Shi, E. Kioupakis, Nano Lett, 2015, 15, 6926 |
11. | B.-C. Qin, Y. Xiao, Y.-M. Zhou, L.-D. Zhao, Rare Met, 2018, 37, 343 |
12. | C. Kamal, A. Chakrabarti, M. Ezawa, Phys. Rev. B, 2016, 93, 125428 |
13. | K. F. Abd El-Rahman, A. A. A. Darwish, E. A. A. El-Shazly, Mater. Sci. Semicond. Process, 2014, 25, 123 |
14. | Y. Xiao, D. Wang, B. Qin, J. Wang, G. Wang, L.-D. Zhao, J. Am. Chem. Soc, 2018, 140, 13097 |
15. | X. Zhang, C. Chang, Y. Zhou, L.-D. Zhao, Materials, 2017, 10, 198 |
16. | S. Liu, X. Guo, M. Li, W. H. Zhang, X. Liu, C. Li, Angew Chem. Int. Ed, 2011, 50, 12050 |
17. | P. C. Wei, S. Bhattacharya, J. He, S. Neeleshwar, R. Podila, Y. Y. Chen, A. M. Rao, Nature, 2016, 539, 452 |
18. | L. D. Zhao, G. Tan, S. Hao, J. He, Y. Pei, H. Chi, H. Wang, S. Gong, H. Xu, V. P. Dravid, C. Uher, G. J. Snyder, C. Wolverton, M. G. Kanatzidis, Science, 2016, 351, 141 |
19. | C. Chang, M. Wu, D. He, Y. Pei, C. F. Wu, X. Wu, H. Yu, F. Zhu, K. Wang, Y. Chen, L. Huang, J. F. Li, J. He, L. D. Zhao, Science, 2018, 360, 778 |
20. | B. Qin, D. Wang, X. Liu, Y. Qin, J. F. Dong, J. Luo, J. W. Li, W. Liu, G. Tan, X. Tang, J. F. Li, J. He, L. D. Zhao, Science, 2021, 373, 556 |
21. | W. He, D. Wang, J.-F. Dong, Y. Qiu, L. Fu, Y. Feng, Y. Hao, G. Wang, J. Wang, C. Liu, J.-F. Li, J. He, L.-D. Zhao, J. Mater. Chem. A, 2018, 6, 10048 |
22. | W. He, D. Wang, H. Wu, Y. Xiao, Y. Zhang, D. He, Y. Feng, Y. J. Hao, J. F. Dong, R. Chetty, L. Hao, D. Chen, J. Qin, Q. Yang, X. Li, J. M. Song, Y. Zhu, W. Xu, C. Niu, X. Li, G. Wang, C. Liu, M. Ohta, S. J. Pennycook, J. He, J. F. Li, L. D. Zhao, Science, 2019, 365, 1418 |
23. | H. Wang, W. Lu, S. Hou, B. Yu, Z. Zhou, Y. Xue, R. Guo, S. Wang, K. Zeng, X. Yan, Nanoscale, 2020, 12, 21913 |
24. | B. Ouyang, C. Chang, L.-D. Zhao, Z. L. Wang, Y. Yang, Nano Energy, 2019, 66, 104111 |
25. | X.-W. Shen, Y.-W. Fang, B.-B. Tian, C.-G. Duan, ACS Appl. Electron. Mater, 2019, 1, 1133 |
26. | Z. Li, L. Sun, Y. Liu, L. Zhu, D. Yu, Y. Wang, Y. Sun, M. Yu, Environ. Sci. :Nano, 2019, 6, 1507 |
27. | C. Liu, S. Zhao, Y. Lu, Y. Chang, D. Xu, Q. Wang, Z. Dai, J. Bao, M. Han, Small, 2017, 13, 1603494 |
28. | M. S. Mahdi, K. Ibrahim, A. Hmood, Naser M. Ahmed, S. A. Azzez, F. I. Mustafa, RSC Adv, 2016, 6, 114980 |
29. | J. Xia, L. Liu, S. Jamil, J. Xie, H. Yan, Y. Yuan, Y. Zhang, S. Nie, J. Pan, X. Wang, G. Cao, Energy Storage Mater, 2019, 17, 1 |
30. | Z. Wang, J. Wang, Y. Zang, Q. Zhang, J. A. Shi, T. Jiang, Y. Gong, C. L. Song, S. H. Ji, L. L. Wang, L. Gu, K. He, W. Duan, X. Ma, X. Chen, Q. K. Xue, Adv. Mater, 2015, 27, 4150 |
31. | W. J. Baumgardner, J. J. Choi, Y. F. Lim, T. Hanrath, J. Am. Chem. Soc, 2010, 132, 9519 |
32. | T. Chattopadhyay, J. Pannetier, H. G. Von Schnering, J. Phys. Chem. Solids, 1986, 47, 879 |
33. | M. J. Peters, L. E. McNeil, Phys. Rev. B, 1990, 41, 5893 |
34. | X. Guan, P. Lu, L. Wu, L. Han, G. Liu, Y. Song, S. Wang, J. Alloys Compd, 2015, 643, 116 |
35. | L.-D. Zhao, C. Chang, G. Tan, M. G. Kanatzidis, Energy Environ. Sci, 2016, 9, 3044 |
36. | A. Rabkin, S. Samuha, R. E. Abutbul, V. Ezersky, L. Meshi, Y. Golan, Nano Lett, 2015, 15, 2174 |
37. | R. E. Abutbul, E. Segev, S. Samuha, L. Zeiri, V. Ezersky, G. Makov, Y. Golan, CrystEngComm, 2016, 18, 1918 |
38. | L. Cheng, Y. Liu, J. Am. Chem. Soc, 2018, 140, 17895 |
39. | G. Schusteritsch, M. Uhrin, C. J. Pickard, Nano Lett, 2016, 16, 2975 |
40. | H. Lang, S. Zhang, Z. Liu, Phys. Rev. B, 2016, 94, 235306 |
41. | X. Liu, D. Wang, H. Wu, J. Wang, Y. Zhang, G. Wang, S. J. Pennycook, L.-D. Zhao, Adv. Funct. Mater, 2019, 29, 1806558 |
42. | G. Shi, E. Kioupakis, J. Appl. Phys, 2015, 117, 065103 |
43. | L. D. Zhao, S. H. Lo, Y. Zhang, H. Sun, G. Tan, C. Uher, C. Wolverton, V. P. Dravid, M. G. Kanatzidis, Nature, 2014, 508, 373 |
44. | S. Wang, J. Yang, T. Toll, J. Yang, W. Zhang, X. Tang, Sci. Rep, 2015, 5, 10136 |
45. | D. Wu, L. Wu, D. He, L.-D. Zhao, W. Li, M. Wu, M. Jin, J. Xu, J. Jiang, L. Huang, Y. Zhu, M. G. Kanatzidis, J. He, Nano Energy, 2017, 35, 321 |
46. | K. Peng, H. Wu, Y. Yan, L. Guo, G. Wang, X. Lu, X. Zhou, J. Mater. Chem. A, 2017, 5, 14053 |
47. | Y.-L. Pei, H. Wu, J. Sui, J. Li, D. Berardan, C. Barreteau, L. Pan, N. Dragoe, W.-S. Liu, J. He, L.-D. Zhao, Energy Environ. Sci, 2013, 6, 1750 |
48. | C.-L. Chen, H. Wang, Y.-Y. Chen, T. Day, G. J. Snyder, J. Mater. Chem. A, 2014, 2, 11171 |
49. | C. Zhou, Y. K. Lee, Y. Yu, S. Byun, Z. Z. Luo, H. Lee, B. Ge, Y. L. Lee, X. Chen, J. Y. Lee, O. Cojocaru-Miredin, H. Chang, J. Im, S. P. Cho, M. Wuttig, V. P. Dravid, M. G. Kanatzidis, I. Chung, Nat. Mater, 2021, 20, 1378 |
50. | Y. K. Lee, Z. Luo, S. P. Cho, M. G. Kanatzidis, I. Chung, Joule, 2019, 3, 719 |
51. | M. A. Franzman, C. W. Schlenker, M. E. Thompson, R. L. Brutchey, J. Am. Chem. Soc, 2010, 132, 4060 |
52. | L. Li, Z. Chen, Y. Hu, X. Wang, T. Zhang, W. Chen, Q. Wang, J. Am. Chem. Soc, 2013, 135, 1213 |
53. | B. Pejova, I. Grozdanov, Thin Solid Films, 2007, 515, 5203 |
54. | Q. Lu, M. Wu, D. Wu, C. Chang, Y. P. Guo, C. S. Zhou, W. Li, X. M. Ma, G. Wang, L. D. Zhao, L. Huang, C. Liu, J. He, Phys. Rev. Lett, 2017, 119, 116401 |
55. | W. Albers, C. Haas, H. J. Vink, J. D. Wasscher, J. Appl. Phys, 1961, 32, 2220 |
56. | K. T. Ramakrishna Reddy, N. Koteswara Reddy, R. W. Miles, Sol. Energy Mater. Sol. Cells, 2006, 90, 3041 |
57. | B. Zhou, S. Li, W. Li, J. Li, X. Zhang, S. Lin, Z. Chen, Y. Pei, ACS Appl. Mater. Interfaces, 2017, 9, 34033 |
58. | H. Wu, X. Lu, G. Wang, K. Peng, H. Chi, B. Zhang, Y. Chen, C. Li, Y. Yan, L. Guo, C. Uher, X. Zhou, X. Han, Adv. Energy Mater, 2018, 8, 1800087 |
59. | G. A. Tritsaris, B. D. Malone, E. Kaxiras, J. Appl. Phys, 2013, 113, 233507 |
60. | C. Xin, J. Zheng, Y. Su, S. Li, B. Zhang, Y. Feng, F. Pan, J. Phys. Chem. C, 2016, 120, 22663 |
61. | C. Gao, H. Shen, L. Sun, Appl. Surf. Sci, 2011, 257, 6750 |
62. | D. Avellaneda, M. T. S. Nair, P. K. Nair, Thin Solid Films, 2009, 517, 2500 |
63. | L.-B. Shi, M. Yang, S. Cao, Q. You, Y.-Y. Niu, Y.-Z. Wang, Appl. Surf. Sci, 2019, 492, 435 |
64. | M. Wu, X. C. Zeng, Nano Lett, 2016, 16, 3236 |
65. | A. Agarwal, M. N. Vashi, D. Lakshminarayana, N. M. Batra, J. Mater. Sci. :Mater. Electron, 2000, 11, 67 |
66. | M. Beekman, G. Cogburn, C. Heideman, S. Rouvimov, P. Zschack, W. Neumann, D. C. Johnson, J. Electron. Mater, 2012, 41, 1476 |
67. | M. Zhou, X. Chen, M. Li, A. Du, J. Mater. Chem. C, 2017, 5, 1247 |
68. | L. Peng, C. Wang, Q. Qian, C. Bi, S. Wang, Y. Huang, ACS Appl. Mater. Interfaces, 2017, 9, 40969 |
69. | Y. Pei, X. Shi, A. LaLonde, H. Wang, L. Chen, G. J. Snyder, Nature, 2011, 473, 66 |
70. | J. P. Heremans, V. Jovovic, E. S. Toberer, A. Saramat, K. Kurosaki, A. Charoenphakdee, S. Yamanaka, G. J. Snyder, Science, 2008, 321, 554 |
71. | W. Liu, X. Tan, K. Yin, H. Liu, X. Tang, J. Shi, Q. Zhang, C. Uher, Phys. Rev. Lett, 2012, 108, 166601 |
72. | K. Biswas, J. He, I. D. Blum, C. I. Wu, T. P. Hogan, D. N. Seidman, V. P. Dravid, M. G. Kanatzidis, Nature, 2012, 489, 414 |
73. | K. F. Hsu, S. Loo, F. Guo, W. Chen, J. S. Dyck, C. Uher, T. Hogan, E. K. Polychroniadis, M. G. Kanatzidis, Science, 2004, 303, 818 |
74. | B. Yu, M. Zebarjadi, H. Wang, K. Lukas, H. Wang, D. Wang, C. Opeil, M. Dresselhaus, G. Chen, Z. Ren, Nano Lett, 2012, 12, 2077 |
75. | Y. L. Pei, H. Wu, D. Wu, F. Zheng, J. He, J. Am. Chem. Soc, 2014, 136, 13902 |
76. | A. T. Duong, V. Q. Nguyen, G. Duvjir, V. T. Duong, S. Kwon, J. Y. Song, J. K. Lee, J. E. Lee, S. Park, T. Min, J. Lee, J. Kim, S. Cho, Nat. Commun, 2016, 7, 13713 |
77. | N. Oka, S. Yamada, T. Yagi, N. Taketoshi, J. Jia, Y. Shigesato, J. Mater. Res, 2014, 29, 1579 |
78. | Q. Tan, J.-F. Li, J. Electron. Mater, 2014, 43, 2435 |
79. | D. Parker, D. J. Singh, J. Appl. Phys, 2010, 108, 083712 |
80. | W. He, T. Hong, D. Wang, X. Gao, L.-D. Zhao, Sci. China Mater, 2021, 64, 3051 |
81. | J. P. Singh, and R. K. Bedi, Jpn. J. Appl. Phys, 1990, 29.5A, L792 |
82. | K. Ananthi, K. Thilakavathy, N. Muthukumarasamy, S. Dhanapandian, K. R. Murali, J. Mater. Sci. :Mater. Electron, 2011, 23, 1338 |
83. | N. Makori, I. Amatalo, P. Karimi, W. Njoroge, J. Phys. Condens. Matter, 2014, 4, 87 |
84. | P. Nair, M. Nair, V. Garcıa, O. Arenas, Y. Pena, A. Castillo, I. Ayala, O. Gomezdaza, A. Sanchez, J. Campos, Sol. Energy Mater. Sol. Cells, 1998, 52, 313 |
85. | D. Lim, H. Suh, M. Suryawanshi, G. Y. Song, J. Y. Cho, J. H. Kim, J. H. Jang, C.-W. Jeon, A. Cho, S. Ahn, J. Heo, Adv. Energy Mater, 2018, 8, 1701605 |
86. | V. E. González-Flores, R. N. Mohan, R. Ballinas-Morales, M. T. S. Nair, P. K. Nair, Thin Solid Films, 2019, 672, 62 |
87. | G. D. Park, J.-K. Lee, Y. C. Kang, Adv. Funct. Mater, 2017, 27, 1603399 |
88. | W. Shi, M. Gao, J. Wei, J. Gao, C. Fan, E. Ashalley, H. Li, Z. Wang, Adv. Sci, 2018, 5, 1700602 |
89. | Y. Xiao, S. H. Lee, Y.-K. Sun, Adv. Energy Mater, 2017, 7, 1601329 |
90. | W. Kang, Y. Wang, J. Xu, J. Mater. Chem. A, 2017, 5, 7667 |
91. | X.-Y. Yu, L. Yu, X. W. D. Lou, Adv. Energy Mater, 2016, 6, 1501333 |
92. | D. Chao, C. Zhu, P. Yang, X. Xia, J. Liu, J. Wang, X. Fan, S. V. Savilov, J. Lin, H. J. Fan, Z. X. Shen, Nat. Commun, 2016, 7, 12122 |
93. | H. Kang, Y. Liu, K. Cao, Y. Zhao, L. Jiao, Y. Wang, H. Yuan, J. Mater. Chem. A, 2015, 3, 17899 |
94. | X. Xu, W. Liu, Y. Kim, J. Cho, Nano Today, 2014, 9, 604 |
95. | L. Wu, H. Lu, L. Xiao, J. Qian, X. Ai, H. Yang, Y. Cao, J. Mater. Chem. A, 2014, 2, 16424 |
96. | W. Ni, B. Wang, J. Cheng, X. Li, Q. Guan, G. Gu, L. Huang, Nanoscale, 2014, 6, 2618 |
97. | M. Zhang, D. Lei, X. Yu, L. Chen, Q. Li, Y. Wang, T. Wang, G. Cao, J. Mater. Chem, 2012, 22, 23091 |
98. | L. Wu, H. Lu, L. Xiao, X. Ai, H. Yang, Y. Cao, J. Power Sources, 2015, 293, 784 |
99. | Y. Li, J. P. Tu, X. H. Huang, H. M. Wu, Y. F. Yuan, Electrochim. Acta, 2006, 52, 1383 |
100. | Y. Li, J. P. Tu, X. H. Huang, H. M. Wu, Y. F. Yuan, Electrochem. Commun, 2007, 9, 49 |
101. | T. Zhou, W. K. Pang, C. Zhang, J. Yang, Z. Chen, H. K. Liu, Z. Guo, ACS Nano, 2014, 8, 8323 |
102. | H.-C. Tao, X.-L. Yang, L.-L. Zhang, S.-B. Ni, J. Electroanal. Chem, 2014, 728, 134 |
103. | S.-C. Zhu, H.-C. Tao, X.-L. Yang, L.-L. Zhang, S.-B. Ni, Ionics, 2015, 21, 2735 |
104. | Y. Zhang, B. Guo, L. Hu, Q. Xu, Y. Li, D. Liu, M. Xu, J. Alloys Compd, 2018, 732, 448 |
105. | B. Zhao, Z. Wang, F. Chen, Y. Yang, Y. Gao, L. Chen, Z. Jiao, L. Cheng, Y. Jiang, ACS Appl. Mater. Interfaces, 2017, 9, 1407 |
106. | S. Li, J. Zheng, Z. Hu, S. Zuo, Z. Wu, P. Yan, F. Pan, RSC Adv, 2015, 5, 72857 |
107. | L. Lu, L. Zhang, H. Zeng, B. Xu, L. Wang, Y. Li, J. Alloys Compd, 2017, 695, 1294 |
108. | P. K. Dutta, U. K. Sen, S. Mitra, RSC Adv, 2014, 4, 43155 |
109. | D. D. Vaughn, 2nd, O. D. Hentz, S. Chen, D. Wang, R. E. Schaak, Chem. Commun, 2012, 48, 5608 |
110. | A. M. Tripathi, S. Mitra, RSC Adv, 2015, 5, 23671 |
111. | J. Cai, Z. Li, P. K. Shen, ACS Appl. Mater. Interfaces, 2012, 4, 4093 |
112. | E. Cho, K. Song, M. H. Park, K. W. Nam, Y. M. Kang, Small, 2016, 12, 2510 |
113. | J.-G. Kang, J.-G. Park, D.-W. Kim, Electrochem. Commun, 2010, 12, 307 |
114. | S. H. Choi, Y. C. Kang, Small, 2014, 10, 474 |
115. | X. Ren, J. Wang, D. Zhu, Q. Li, W. Tian, L. Wang, J. Zhang, L. Miao, P. K. Chu, K. Huo, Nano Energy, 2018, 54, 322 |
116. | X. Xiong, C. Yang, G. Wang, Y. Lin, X. Ou, J.-H. Wang, B. Zhao, M. Liu, Z. Lin, K. Huang, Energy Environ. Sci, 2017, 10, 1757 |
117. | J. Yan, Z. Fan, W. Sun, G. Ning, T. Wei, Q. Zhang, R. Zhang, L. Zhi, F. Wei, Adv. Funct. Mater, 2012, 22, 2632 |
118. | S. Boukhalfa, K. Evanoff, G. Yushin, Energy Environ. Sci, 2012, 5, 6872 |
119. | Q. Li, Z. L. Wang, G. R. Li, R. Guo, L. X. Ding, Y. X. Tong, Nano Lett, 2012, 12, 3803 |
120. | A. Izadi-Najafabadi, S. Yasuda, K. Kobashi, T. Yamada, D. N. Futaba, H. Hatori, M. Yumura, S. Iijima, K. Hata, Adv. Mater, 2010, 22, E235 |
121. | J. W. Lee, A. S. Hall, J.-D. Kim, T. E. Mallouk, Chem. Mater, 2012, 24, 1158 |
122. | Z. S. Wu, W. Ren, D. W. Wang, F. Li, B. Liu, H. M. Cheng, ACS Nano, 2010, 4, 5835 |
123. | B. C. Kim, J.-Y. Hong, G. G. Wallace, H. S. Park, Adv. Energy Mater, 2015, 5, 1500959 |
124. | G. Wang, L. Zhang, J. Zhang, Chem. Soc. Rev, 2012, 41, 797 |
125. | X. Lu, T. Zhai, X. Zhang, Y. Shen, L. Yuan, B. Hu, L. Gong, J. Chen, Y. Gao, J. Zhou, Y. Tong, Z. L. Wang, Adv. Mater, 2012, 24, 938 |
126. | X. Cao, B. Zheng, W. Shi, J. Yang, Z. Fan, Z. Luo, X. Rui, B. Chen, Q. Yan, H. Zhang, Adv. Mater, 2015, 27, 4695 |
127. | P. Yang, Y. Ding, Z. Lin, Z. Chen, Y. Li, P. Qiang, M. Ebrahimi, W. Mai, C. P. Wong, Z. L. Wang, Nano Lett, 2014, 14, 731 |
128. | L. Li, S. Peng, H. B. Wu, L. Yu, S. Madhavi, X. W. D. Lou, Adv. Energy Mater, 2015, 5, 1500360 |
129. | J. Xu, Q. Wang, X. Wang, Q. Xiang, B. Liang, D. Chen, G. Shen, ACS Nano, 2013, 7, 5453 |
130. | J. Feng, X. Sun, C. Wu, L. Peng, C. Lin, S. Hu, J. Yang, Y. Xie, J. Am. Chem. Soc, 2011, 133, 17832 |
131. | M. Acerce, D. Voiry, M. Chhowalla, Nat. Nanotechnol, 2015, 10, 313 |
132. | N. Choudhary, M. Patel, Y.-H. Ho, N. B. Dahotre, W. Lee, J. Y. Hwang, W. Choi, J. Mater. Chem. A, 2015, 3, 24049 |
133. | L. Cao, S. Yang, W. Gao, Z. Liu, Y. Gong, L. Ma, G. Shi, S. Lei, Y. Zhang, S. Zhang, R. Vajtai, P. M. Ajayan, Small, 2013, 9, 2905 |
134. | L. Liu, Y. Yu, C. Yan, K. Li, Z. Zheng, Nat. Commun, 2015, 6, 7260 |
135. | B. Xie, C. Yang, Z. Zhang, P. Zou, Z. Lin, G. Shi, Q. Yang, F. Kang, C. P. Wong, ACS Nano, 2015, 9, 5636 |
136. | K. Wang, Q. Meng, Y. Zhang, Z. Wei, M. Miao, Adv. Mater, 2013, 25, 1494 |
137. | M. Ghidiu, M. R. Lukatskaya, M. Q. Zhao, Y. Gogotsi, M. W. Barsoum, Nature, 2014, 516, 78 |
138. | L. Kou, T. Huang, B. Zheng, Y. Han, X. Zhao, K. Gopalsamy, H. Sun, C. Gao, Nat. Commun, 2014, 5, 3754 |
139. | D. Ni, Y. Chen, X. Yang, C. Liu, K. Cai, J. Alloys Compd, 2018, 737, 623 |
140. | C. Zhang, H. Yin, M. Han, Z. Dai, H. Pang, Y. Zheng, Y. Q. Lan, J. Bao, J. Zhu, ACS Nano, 2014, 8, 3761 |
141. | H. Chauhan, M. K. Singh, S. A. Hashmi, S. Deka, RSC Adv, 2015, 5, 17228 |
142. | M. K. Kim, J. S. Lee, ACS Nano, 2018, 12, 1680 |
143. | M. Prezioso, F. Merrikh-Bayat, B. D. Hoskins, G. C. Adam, K. K. Likharev, D. B. Strukov, Nature, 2015, 521, 61 |
144. | X. Yan, Q. Zhao, A. P. Chen, J. Zhao, Z. Zhou, J. Wang, H. Wang, L. Zhang, X. Li, Z. Xiao, K. Wang, C. Qin, G. Wang, Y. Pei, H. Li, D. Ren, J. Chen, Q. Liu, Small, 2019, 15, 1901423 |
145. | G. S. Park, Y. B. Kim, S. Y. Park, X. S. Li, S. Heo, M. J. Lee, M. Chang, J. H. Kwon, M. Kim, U. I. Chung, R. Dittmann, R. Waser, K. Kim, Nat. Commun, 2013, 4, 2382 |
146. | M. Hu, C. E. Graves, C. Li, Y. Li, N. Ge, E. Montgomery, N. Davila, H. Jiang, R. S. Williams, J. J. Yang, Q. Xia, J. P. Strachan, Adv. Mater, 2018, 30, 1705914 |
147. | Z. R. Wang, S. Joshi, S. E. Savel'ev, H. Jiang, R. Midya, P. Lin, M. Hu, N. Ge, J. P. Strachan, Z. Y. Li, Q. Wu, M. Barne, G. L. Li, H. L. Xin, R. S. Williams, Q. F. Xia, J. J. Yang, Nat. Mater, 2017, 16, 101 |
148. | J. van den Hurk, A. C. Dippel, D. Y. Cho, J. Straquadine, U. Breuer, P. Walter, R. Waser, I. Valov, Phys. Chem. Chem. Phys, 2014, 16, 18217 |
149. | H. Tian, X. Cao, Y. Xie, X. Yan, A. Kostelec, D. DiMarzio, C. Chang, L. D. Zhao, W. Wu, J. Tice, J. J. Cha, J. Guo, H. Wang, ACS Nano, 2017, 11, 7156 |
150. | H. Tian, X. F. Wang, M. A. Mohammad, G. Y. Gou, F. Wu, Y. Yang, T. L. Ren, Nat. Commun, 2018, 9, 4305 |
151. | H. Wang, X. Yan, S. Wang, N. Lu, ACS Appl. Mater. Interfaces, 2021, 13, 17844 |
152. | H. Wang, T. Yu, J. Zhao, S. Wang, X. Yan, Sci. China Mater, 2021, 64, 1989 |
153. | Y. Yan, S. Krishnakumar, H. Yu, S. Ramishetti, L. W. Deng, S. Wang, L. Huang, D. Huang, J. Am. Chem. Soc, 2013, 135, 5312 |
154. | R. Atkinson, Atmos. Environ, 2000, 34, 2063 |
155. | M. Penza, C. Martucci, G. Cassano, Sens. Actuators, B, 1998, 50, 52 |
156. | D. H. Nguyen, S. A. El-Safty, Chemistry, 2011, 17, 12896 |
157. | N. Tammanoon, A. Wisitsoraat, C. Sriprachuabwong, D. Phokharatkul, A. Tuantranont, S. Phanichphant, C. Liewhiran, ACS Appl. Mater. Interfaces, 2015, 7, 24338 |
158. | S. Xu, J. Gao, L. Wang, K. Kan, Y. Xie, P. Shen, L. Li, K. Shi, Nanoscale, 2015, 7, 14643 |
159. | Y. Han, D. Huang, Y. Ma, G. He, J. Hu, J. Zhang, N. Hu, Y. Su, Z. Zhou, Y. Zhang, Z. Yang, ACS Appl. Mater. Interfaces, 2018, 10, 22640 |
160. | J. Hao, D. Zhang, Q. Sun, S. Zheng, J. Sun, Y. Wang, Nanoscale, 2018, 10, 7210 |
161. | D. Wang, K. Wan, M. Zhang, H. Li, P. Wang, X. Wang, J. Yang, Sens. Actuators, B, 2019, 283, 714 |
162. | F. Schedin, A. K. Geim, S. V. Morozov, E. W. Hill, P. Blake, M. I. Katsnelson, K. S. Novoselov, Nat. Mater, 2007, 6, 652 |
163. | L. Kou, T. Frauenheim, C. Chen, J. Phys. Chem. Lett, 2014, 5, 2675 |
164. | Q. Yang, X.-P. Chen, R.-S. Meng, J.-K. Jiang, Q.-H. Liang, C.-J. Tan, M. Cai, X. Sun, D.-G. Yang, T.-L. Ren, IEEE Electron Device Lett, 2016, 37, 660 |
165. | G. Neri, Chemosensors, 2017, 5, 21 |
166. | F. Ricciardella, S. Vollebregt, T. Polichetti, M. Miscuglio, B. Alfano, M. L. Miglietta, E. Massera, G. Di Francia, P. M. Sarro, Nanoscale, 2017, 9, 6085 |
167. | S. Yang, C. Jiang, S.-h. Wei, Appl. Phys. Rev, 2017, 4, 021304 |
168. | N. Gillgren, D. Wickramaratne, Y. Shi, T. Espiritu, J. Yang, J. Hu, J. Wei, X. Liu, Z. Mao, K. Watanabe, T. Taniguchi, M. Bockrath, Y. Barlas, R. K. Lake, C. Ning Lau, 2D Mater, 2014, 2, 011001 |
169. | S. Guo, L. Yuan, X. Liu, W. Zhou, X. Song, S. Zhang, Chem. Phys. Lett, 2017, 686, 83 |
170. | F.-F. Hu, H.-Y. Tang, C.-J. Tan, H.-Y. Ye, X.-P. Chen, G.-Q. Zhang, IEEE Electron Device Lett, 2017, 38, 983 |
171. | J. Wang, G.-F. Yang, J.-J. Xue, J.-M. Lei, D.-J. Chen, H. Lu, R. Zhang, Y.-D. Zheng, IEEE Electron Device Lett, 2018, 39, 599 |
172. | H. Ye, L. Liu, Y. Xu, L. Wang, X. Chen, K. Zhang, Y. Liu, S. W. Koh, G. Zhang, Appl. Surf. Sci, 2019, 484, 33 |
173. | X. Wang, Y. Liu, J. Dai, Q. Chen, X. Huang, W. Huang, Chemistry, 2020, 26, 3870 |
174. | Q. Sun, J. Wang, J. Hao, S. Zheng, P. Wan, T. Wang, H. Fang, Y. Wang, Nanoscale, 2019, 11, 13741 |
175. | M. F. Afsar, M. A. Rafiq, A. I. Y. Tok, RSC Adv, 2017, 7, 21556 |
176. | B. Parveen, M. Hassan, S. Atiq, S. Riaz, S. Naseem, M. A. Toseef, Prog. Nat. Sci. :Mater. Int, 2017, 27, 303 |
177. | Z. Wen, C. Li, D. Wu, A. Li, N. Ming, Nat. Mater, 2013, 12, 617 |
178. | J. Junquera, P. Ghosez, Nature, 2003, 422, 506 |
179. | P. Z. Wang, T. Y. Cai, S. Ju, Y. Z. Wu, Sci. Rep, 2016, 6, 24209 |
180. | L. L. Tao, J. Wang, Appl. Phys. Lett, 2016, 108, 062903 |
181. | K. Klyukin, L. L. Tao, E. Y. Tsymbal, V. Alexandrov, Phys. Rev. Lett, 2018, 121, 056601 |
182. | R. Fei, W. Kang, L. Yang, Phys. Rev. Lett, 2016, 117, 097601 |
183. | W. Ding, J. Zhu, Z. Wang, Y. Gao, D. Xiao, Y. Gu, Z. Zhang, W. Zhu, Nat. Commun, 2017, 8, 14956 |
184. | A. I. Lebedev, J. Appl. Phys, 2018, 124, 164302 |
185. | F. Xiong, X. Zhang, Z. Lin, Y. Chen, J. Materiomics, 2018, 4, 139 |
186. | L. Kang, P. Jiang, N. Cao, H. Hao, X. Zheng, L. Zhang, Z. Zeng, Nanoscale, 2019, 11, 16837 |
187. | C. Cui, W. J. Hu, X. Yan, C. Addiego, W. Gao, Y. Wang, Z. Wang, L. Li, Y. Cheng, P. Li, X. Zhang, H. N. Alshareef, T. Wu, W. Zhu, X. Pan, L. J. Li, Nano Lett, 2018, 18, 1253 |
188. | J. Lu, W. Luo, J. Feng, H. Xiang, Nano Lett, 2018, 18, 595 |
189. | K. A. Campbell, C. M. Anderson, Microelectron. J, 2007, 38, 52 |
190. | F. H. Koppens, T. Mueller, P. Avouris, A. C. Ferrari, M. S. Vitiello, M. Polini, Nat. Nanotechnol, 2014, 9, 780 |
191. | K. F. Mak, J. Shan, Nat. Photonics, 2016, 10, 216 |
192. | C. Yan, L. Gan, X. Zhou, J. Guo, W. Huang, J. Huang, B. Jin, J. Xiong, T. Zhai, Y. Li, Adv. Funct. Mater, 2017, 27, 1702918 |
193. | F. Xia, T. Mueller, Y.-m. Lin, A. Valdes-Garcia, P. Avouris, Nat. Nanotechnol, 2009, 4, 839 |
194. | X. Zhou, X. Hu, S. Zhou, H. Song, Q. Zhang, L. Pi, L. Li, H. Li, J. Lu, T. Zhai, Adv. Mater, 2018, 30, 1703286 |
195. | O. Lopez-Sanchez, D. Lembke, M. Kayci, A. Radenovic, A. Kis, Nat. Nanotechnol, 2013, 8, 497 |
196. | Z. Wang, Q. Li, F. Besenbacher, M. Dong, Adv. Mater, 2016, 28, 10224 |
197. | F. W. Wise, Acc Chem. Res, 2000, 33, 773 |
198. | I. Moreels, Y. Justo, B. De Geyter, K. Haustraete, J. C. Martins, Z. Hens, ACS Nano, 2011, 5, 2004 |
199. | L. Hao, Y. Du, Z. Wang, Y. Wu, H. Xu, S. Dong, H. Liu, Y. Liu, Q. Xue, Z. Han, K. Yan, M. Dong, Nanoscale, 2020, 12, 7358 |
200. | S. Yuan, Y. H. Zhu, W. Li, S. Wang, D. Xu, L. Li, Y. Zhang, X. B. Zhang, Adv. Mater, 2017, 29, 1602469 |
201. | X. Liu, Y. Li, B. Zhou, X. Wang, A. N. Cartwright, M. T. Swihart, Chem. Mater, 2014, 26, 3515 |
202. | J. Ning, G. Xiao, T. Jiang, L. Wang, Q. Dai, B. Zou, B. Liu, Y. Wei, G. Chen, G. Zou, CrystEngComm, 2011, 13, 4161 |
203. | D. D. Vaughn, 2nd, S. I. In, R. E. Schaak, ACS Nano, 2011, 5, 8852 |
204. | L. Zhao, M. Yosef, M. Steinhart, P. Goring, H. Hofmeister, U. Gosele, S. Schlecht, Angew. Chem. Int. Ed, 2005, 45, 311 |
205. | H. Qiao, Z. Huang, X. Ren, H. Yao, S. Luo, P. Tang, X. Qi, J. Zhong, J. Mater. Sci, 2018, 53, 4371 |
206. | K. Patel, P. Chauhan, A. B. Patel, G. K. Solanki, K. D. Patel, V. M. Pathak, ACS Appl. Nano Mater, 2020, 3, 11143 |
207. | M. Kumar, S. Rani, P. Vashistha, A. Pandey, G. Gupta, S. Husale, V. N. Singh, J. Alloys Compd, 2021, 879, 160307 |
208. | Y. Zhong, L. Zhang, V. Linseis, B. Qin, W. Chen, L.-D. Zhao, H. Zhu, Nano Energy, 2020, 72, 104742 |
209. | D. Zheng, H. Fang, M. Long, F. Wu, P. Wang, F. Gong, X. Wu, J. C. Ho, L. Liao, W. Hu, ACS Nano, 2018, 12, 7239 |
210. | A. S. Pawbake, S. R. Jadkar, D. J. Late, Mater. Res. Express, 2016, 3, 105038 |
211. | J. Yao, Z. Zheng, G. Yang, Adv. Funct. Mater, 2017, 27, 1603399 |
212. | F. Jamali-Sheini, M. Cheraghizade, L. Heshmatynezhad, Semicond. Sci. Technol, 2019, 34, 045008 |
213. | H. Yao, S. Luo, G. S. Duesberg, X. Qi, D. Lu, C. Yue, J. Zhong, AIP Adv, 2018, 8, 075123 |
214. | Z. Jia, J. Xiang, F. Wen, R. Yang, C. Hao, Z. Liu, ACS Appl. Mater. Interfaces, 2016, 8, 4781 |
215. | J. Liu, Q. Huang, K. Zhang, Y. Xu, M. Guo, Y. Qian, Z. Huang, F. Lai, L. Lin, Nanoscale Res. Lett, 2017, 12, 259 |
216. | Y. Zhong, L. Zhang, M. Sun, M. Wang, W. Chen, S. Lin, D. Xie, H. Zhu, Mater. Today Energy, 2019, 12, 418 |
217. | M. Amirmazlaghani, F. Raissi, IEEE Trans. Device Mater. Reliab, 2018, 18, 429 |
218. | H. Tian, C. Fan, G. Liu, S. Yuan, Y. Zhang, M. Wang, E. Li, Appl. Surf. Sci, 2019, 487, 1043 |
219. | Z. Wang, R. Yu, C. Pan, Z. Li, J. Yang, F. Yi, Z. L. Wang, Nat. Commun, 2015, 6, 8401 |
220. | Y. Dai, X. Wang, W. Peng, C. Xu, C. Wu, K. Dong, R. Liu, Z. L. Wang, Adv. Mater, 2018, 30, 1705893 |
221. | B. Ouyang, K. Zhang, Y. Yang, iScience, 2018, 1, 16 |
222. | H. Xu, L. Hao, H. Liu, S. Dong, Y. Wu, Y. Liu, B. Cao, Z. Wang, C. Ling, S. Li, Z. Xu, Q. Xue, K. Yan, ACS Appl. Mater. Interfaces, 2020, 12, 35250 |
223. | N. M. Gabor, J. C. Song, Q. Ma, N. L. Nair, T. Taychatanapat, K. Watanabe, T. Taniguchi, L. S. Levitov, P. Jarillo-Herrero, Science, 2011, 334, 648 |
224. | X. Wang, Y. Dai, R. Liu, X. He, S. Li, Z. L. Wang, ACS Nano, 2017, 11, 8339 |
225. | X. Cai, A. B. Sushkov, R. J. Suess, M. M. Jadidi, G. S. Jenkins, L. O. Nyakiti, R. L. Myers-Ward, S. Li, J. Yan, D. K. Gaskill, T. E. Murphy, H. D. Drew, M. S. Fuhrer, Nat. Nanotechnol, 2014, 9, 814 |
226. | Y. Dai, X. Wang, W. Peng, H. Zou, R. Yu, Y. Ding, C. Wu, Z. L. Wang, ACS Nano, 2017, 11, 7118 |
227. | H. Zou, X. Li, W. Peng, W. Wu, R. Yu, C. Wu, W. Ding, F. Hu, R. Liu, Y. Zi, Z. L. Wang, Adv. Mater, 2017, 29, 1701412 |
228. | Z. Wang, R. Yu, C. Pan, Y. Liu, Y. Ding, Z. L. Wang, Adv. Mater, 2015, 27, 1553 |
229. | K. Zhao, B. Ouyang, Y. Yang, iScience, 2018, 3, 208 |
230. | K. Zhao, B. Ouyang, C. R. Bowen, Z. L. Wang, Y. Yang, Nano Energy, 2020, 71, 104632 |
231. | B. Ouyang, W. He, L. Wu, L.-D. Zhao, Y. Yang, Nano Energy, 2021, 88, 106268 |
232. | K. Sunada, Y. Kikuchi, K. Hashimoto, A. Fujishima, Environ. Sci. Technol, 1998, 32, 726 |
233. | A. Fujishima, K. Honda, Nature, 1972, 238, 37 |
234. | Y. Ohko, K. Hashimoto, A. Fujishima, J. Phys. Chem. A, 1997, 101, 8057 |
235. | R. Shi, G. Huang, J. Lin, Y. Zhu, Phys. Chem. C, 2009, 113, 19633 |
236. | C. Yang, W. Wang, Z. Shan, F. Huang, J. Solid State Chem, 2009, 182, 807 |
237. | X. Hu, G. Song, W. Li, Y. Peng, L. Jiang, Y. Xue, Q. Liu, Z. Chen, J. Hu, Mater. Res. Bull, 2013, 48, 2325 |
238. | K. Yao, J. Li, S. Shan, Q. Jia, Catal. Commun, 2017, 101, 51 |
239. | A. G. Shiravizadeh, R. Yousefi, S. M. Elahi, S. A. Sebt, Phys. Chem. Chem. Phys, 2017, 19, 18089 |
240. | R. Tang, H. Su, Y. Sun, X. Zhang, L. Li, C. Liu, S. Zeng, D. Sun, J. Colloid Interface Sci, 2016, 466, 388 |
241. | Q. Xia, B. Huang, X. Yuan, H. Wang, Z. Wu, L. Jiang, T. Xiong, J. Zhang, G. Zeng, H. Wang, J. Colloid Interface Sci, 2018, 530, 481 |
242. | Z. Li, X. Meng, Z. Zhang, J. Colloid Interface Sci, 2019, 537, 345 |
243. | L. Fu, Phys. Rev. Lett, 2011, 106, 106802 |
244. | T. H. Hsieh, H. Lin, J. Liu, W. Duan, A. Bansil, L. Fu, Nat. Commun, 2012, 3, 982 |
245. | J. Liu, W. Duan, L. Fu, Phys. Rev. B, 2013, 88, 241303 |
246. | Y. Tanaka, Z. Ren, T. Sato, K. Nakayama, S. Souma, T. Takahashi, K. Segawa, Y. Ando, Nat. Phys, 2012, 8, 800 |
247. | S. Y. Xu, C. Liu, N. Alidoust, M. Neupane, D. Qian, I. Belopolski, J. D. Denlinger, Y. J. Wang, H. Lin, L. A. Wray, G. Landolt, B. Slomski, J. H. Dil, A. Marcinkova, E. Morosan, Q. Gibson, R. Sankar, F. C. Chou, R. J. Cava, A. Bansil, M. Z. Hasan, Nat. Commun, 2012, 3, 1192 |
248. | P. Dziawa, B. J. Kowalski, K. Dybko, R. Buczko, A. Szczerbakow, M. Szot, E. Lusakowska, T. Balasubramanian, B. M. Wojek, M. H. Berntsen, O. Tjernberg, T. Story, Nat. Mater, 2012, 11, 1023 |
249. | P. Barone, D. Di Sante, S. Picozzi, Phys. Status Solidi RRL, 2013, 7, 1102 |
250. | Y. Sun, Z. Zhong, T. Shirakawa, C. Franchini, D. Li, Y. Li, S. Yunoki, X.-Q. Chen, Phys. Rev. B, 2013, 88, 235122 |
251. | J. Xia, Y. Yuan, H. Yan, J. Liu, Y. Zhang, L. Liu, S. Zhang, W. Li, X. Yang, H. Shu, X. Wang, G. Cao, J. Power Sources, 2020, 449, 227559 |
252. | K. Liang, L. Ju, S. Koul, A. Kushima, Y. Yang, Adv. Energy Mater, 2019, 9, 1802543 |
253. | X. Zhou, L. Gan, Q. Zhang, X. Xiong, H. Li, Z. Zhong, J. Han, T. Zhai, J. Mater. Chem. C, 2016, 4, 2111 |
254. | G. Mohan Kumar, X. Fu, P. Ilanchezhiyan, S. U. Yuldashev, D. J. Lee, H. D. Cho, T. W. Kang, ACS Appl. Mater. Interfaces, 2017, 9, 32142 |
255. | J. Chao, Z. Wang, X. Xu, Q. Xiang, W. Song, G. Chen, J. Hu, D. Chen, RSC Adv, 2013, 3, 2746 |
256. | J. Xu, Y. Yang, Z. Xie, J Mater. Sci. :Mater. Electron, 2014, 25, 3028 |
257. | D. Yin, C. Dun, X. Gao, Y. Liu, X. Zhang, D. L. Carroll, M. T. Swihart, Small, 2018, 14, 1801949 |
258. | N. A. Rongione, M. Li, H. Wu, H. D. Nguyen, J. S. Kang, B. Ouyang, H. Xia, Y. Hu, Adv. Electron. Mater, 2019, 5, 1800774 |
259. | X. Liu, Y. Du, Q. Meng, Y. Dou, M. Jin, J. Xu, S. Z. Shen, Adv. Eng. Mater, 2020, 22, 2000605 |
260. | J. Zhang, T. Zhang, H. Zhang, Z. Wang, C. Li, Z. Wang, K. Li, X. Huang, M. Chen, Z. Chen, Z. Tian, H. Chen, L. D. Zhao, L. Wei, Adv. Mater, 2020, 32, 2002702 |
This is an open access article under the terms of the Creative Commons Attribution License, which permits use, distribution and reproduction in any medium, provided the original work is properly cited.
Schematic of the multifunctional applications of SnSe/SnS beyond thermoelectricity, typically including photodetector, solar cell, photocatalysis, topological insulator, gas sensor, ion battery, flexibility, and memristor, etc.[23-30]
Schematic of the Pnma and Cmcm crystal structures of SnSe/SnS, the claret ball represents Sn atom and the orange ball represents Se(S) atom. (a) Crystal structure of Pnma phase along b-axis; (b) Crystal structure of Cmcm phase along b-axis; (c) SnSe7/SnS7 3D structure, the dotted line represents the weak covalent bonding.
(a) Structure diagram of SnSe energy band. Red solid and dash lines represent the positions of Fermi level with different carrier concentrations. Reproduced with permission.[18] Copyright 2016, American Association for the Advancement of Science; (b) Angle resolved photoelectron spectroscopy (ARPES) band dispersion of VBM1 and VBM2 along Γ-Z and Γ-Y directions. Reproduced with permission.[54] Copyright 2017, American Physical Society; The energy band structures of SnSe with different number of layers. (c) single-layer; (d) double-layer; and (e) bulk SnSe. Reproduced with permission.[10] Copyright 2015, American Chemical Society.
(a) Schematic of SnS Pnma energy band structures. The number on the energy band diagram represents the maximum value of the valence band (1, 2, 3, 4). The horizontal lines of different colors represent the position of Fermi level when the carrier concentration is ~ 9 × 1017 cm−3, ~ 5 × 1019 cm−3, ~ 9 × 1019 cm−3, ~ 4 × 1020 cm−3, respectively. Reproduced with permission.[21] Copyright 2018, Royal Society of Chemistry; (b) Brillouin zone of SnS and high symmetry points; (c) Angle resolved photoelectron spectroscopy (ARPES) band structure along the X-U direction; (d) ARPES band structures of SnS along the Γ-Y, Γ-Z, and X-U directions. Three parts show the band dispersion of the three VBMs in SnS. Reproduced with permission.[22] Copyright 2019, American Association for the Advancement of Science.
(a) Schematic of multivariate valence band of SnSe. Reproduced with permission.[35] Copyright 2016, Royal Society of Chemistry; (b) Schematic illustration of multiband synglisis in SnSe. Reproduced with permission.[20] Copyright 2021, American Association for the Advancement of Science; (c) Schematic 3D-charge and 2D-phonon transports in n-type SnSe, the p-type SnSe is also plotted for comparison. The colored dots represent the charge densities. The gray blocks represent the two-atom-thick SnSe slabs along the out-of-plane direction (a-axis) of SnSe. Reproduced with permission.[19] Copyright 2018, American Association for the Advancement of Science; (d) Schematic diagram of variation trend of three valence bands with the increase of temperature for SnS. Reproduced with permission.[22] Copyright 2019, American Association for the Advancement of Science.
(a) Schematic of SnSe-based solid solar cell device structure, with SnSe film as absorption layer and CdS as n-type buffer layer; (b) Schematic of cross-sectional FESEM (field emission scanning electron microscope) image of a complete SnS-based solar cell device. Reproduced with permission.[8] Copyright 2019, Wiley-VCH.
(a) Schematic illustration of the vertical SnSe nanoplates on N-doped graphite. Reproduced with permission.[115] Copyright 2018, Elsevier; (b) Stereoscopic diagram of SnS/3D N-doped graphene hybrid anode material, in which orange spheroids represent SnS and gray lamellar structures represent 3D N-doped graphene. Reproduced with permission.[116] Copyright 2017, Royal Society of Chemistry.
(a) Diagram of the SnSe synthesized by the microwave-assisted synthesis. The Sn raw material solution and Se raw material solution are mixed and stirred, and then heated by microwave for 15min. After cleaning, SnSe was dried in vacuum. Reproduced with permission.[139] Copyright 2018, Elsevier; (b) Schematic of 3D porous SnS/S-doped graphene hybrid nanoarchitectures (HNAs). Reproduced with permission.[27] Copyright 2017, Wiley-VCH.
(a) Schematics of black phosphorus-SnSe heterojunction synaptic device. Presynaptic input is applied at the silicon bottom gate terminal. The electrode in contact with SnSe is grounded. Postsynaptic output is measured on electrodes in contact with black phosphorus. Vbias is applied between black phosphorus and SnSe, and voltage Vg is applied between input terminal and SnSe; (b) Schematics of biological synapses that can jointly release excitatory and inhibitory neurotransmitters. Reproduced with permission.[149] Copyright 2017, American Chemical Society; (c) Schematics of the resistance switching mechanism for the SnSe-based memristor devices in their initial, high-resistance, and low-resistance states. Reproduced with permission.[152] Copyright 2021, Science China Press and Springer-Verlag GmbH, Germany.
(a) Schematic of the sensing principle of SnSe(50%)/SnSe2(50%) heterostructures was responsed to NO2 at room temperature under laser illumination; (b) Schematic of the band structure of SnSe(50%)/SnSe2(50%) in N2 or NO2 atmosphere. Evac, EF, EC and EV denote the energy level for vacuum, Fermi level, conduction band and valence band, respectively. Reproduced with permission.[173] Copyright 2020, Chemistry Europe.
(a) Schematic of the 2D ferroelectric tunnel junction device based on homostructure. The pristine SnSe semiconductor with in-plane ferroelectricity acts as a ferroelectric barrier. The electrodes on both sides are p-type and n-type SnSe respectively; (b) Switching mechanisms of the 2D ferroelectric tunnel junction p-SC/FE/n-SC (SC represents single crystal and FE represents ferroelectric barrier, and P+x and P-x represent the polarization direction). The black “+” and “−” symbols in the FE area represent positive and negative charges, respectively. The red “+” in p-SC and blue “−” in n-SC electrodes represent hole and electrons, respectively. The “⊕” and “⊖” represent ionized donors and acceptors, respectively; (c) Overall potential energy profiles with corresponding band diagrams. The barrier width in either P+x or P−x state is given by the green line. The average potential barrier height is showed by the orange line. Reproduced with permission. [25] Copyright 2019, American Chemical Society.
(a) Schematic of photodetector structure; (b, c) Schematic working state and energy band structure under the light illumination condition; (d, e) Schematic working state and energy band structure under the cooling condition; (f, g) Schematic working state and energy band structure under the combined action of light illumination and cooling. Reproduced with permission.[24] Copyright 2019, Elsevier.
Schematic of the synthesis of the SnSe-SnO2 core–shell nanocomposites and photothermal and photocatalytic mechanisms of degradation of sewage under light conditions. Reproduced with permission.[26] Copyright 2019, Royal Society of Chemistry. During operation, light promotes the generation of electrons and holes, which are transferred to the surfaces of the materials and catalyze the reactions with the surrounding substances. And the unreacted electrons will transfer from the conduction band to the valence band and release heat, producing photothermal effect.
(a) The bulk and (111) surface Brillouin zones of SnSe; (b-e) The energy band structures of a 16 nm SnSe (111) film. The bandmap around
(a) Schematic diagram of the thermoelectric generators based on SnSe films. Reproduced with permission.[208] Copyright 2020, Elsevier; (b) Schematic diagram of SnSe films under mechanical bending. Reproduced with permission.[258] Copyright 2019, Wiley-VCH; (c) Schematic diagram of thermal drawing SnSe fibers and post-draw laser recrystallization process. The insets show the cross-sectional microscope image of SnSe fibers and the crystal structure